Cephalopod
A cephalopod (/ˈsɛfələpɒd, ˈkɛf-/) is any member of the molluscan class Cephalopoda (Greek plural κεφαλόποδες, kephalópodes; "head-feet")[2] such as a squid, octopus, cuttlefish, or nautilus. These exclusively marine animals are characterized by bilateral body symmetry, a prominent head, and a set of arms or tentacles (muscular hydrostats) modified from the primitive molluscan foot. Fishermen sometimes call cephalopods "inkfish," referring to their common ability to squirt ink. The study of cephalopods is a branch of malacology known as teuthology.
Cephalopod | |
---|---|
![]() | |
Bigfin reef squid (Sepioteuthis lessoniana) | |
Scientific classification ![]() | |
Kingdom: | Animalia |
Phylum: | Mollusca |
Class: | Cephalopoda Cuvier, 1797 |
Subclasses | |
Cephalopods became dominant during the Ordovician period, represented by primitive nautiloids. The class now contains two, only distantly related, extant subclasses: Coleoidea, which includes octopuses, squid, and cuttlefish; and Nautiloidea, represented by Nautilus and Allonautilus. In the Coleoidea, the molluscan shell has been internalized or is absent, whereas in the Nautiloidea, the external shell remains. About 800 living species of cephalopods have been identified. Two important extinct taxa are the Ammonoidea (ammonites) and Belemnoidea (belemnites).
Distribution
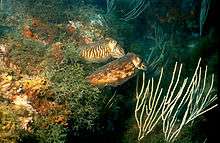
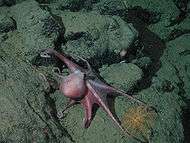
Right: Benthoctopus sp. on the Davidson Seamount at 2,422 m depth
There are over 800 extant species of cephalopod,[3] although new species continue to be described. An estimated 11,000 extinct taxa have been described, although the soft-bodied nature of cephalopods means they are not easily fossilised.[4]
Cephalopods are found in all the oceans of Earth. None of them can tolerate freshwater, but the brief squid, Lolliguncula brevis, found in Chesapeake Bay, is a notable partial exception in that it tolerates brackish water.[5] Cephalopods are thought to be unable to live in freshwater due to multiple biochemical constraints, and in their 400+ million year existence have never ventured into fully freshwater habitats.[6]
Cephalopods occupy most of the depth of the ocean, from the abyssal plain to the sea surface. Their diversity is greatest near the equator (~40 species retrieved in nets at 11°N by a diversity study) and decreases towards the poles (~5 species captured at 60°N).[7]
Biology
Nervous system and behavior
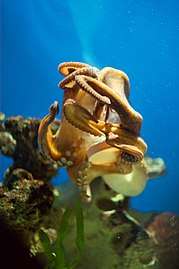
_behavior.jpg)
Right: Hawaiian bobtail squid, Euprymna scolopes, burying itself in the sand, leaving only the eyes exposed
Cephalopods are widely regarded as the most intelligent of the invertebrates, and have well developed senses and large brains (larger than those of gastropods).[8] The nervous system of cephalopods is the most complex of the invertebrates[9][10] and their brain-to-body-mass ratio falls between that of endothermic and ectothermic vertebrates.[7]:14 Captive cephalopods have also been known to climb out of their aquaria, maneuver a distance of the lab floor, enter another aquarium to feed on the crabs, and return to their own aquarium.[11]
The brain is protected in a cartilaginous cranium. The giant nerve fibers of the cephalopod mantle have been widely used for many years as experimental material in neurophysiology; their large diameter (due to lack of myelination) makes them relatively easy to study compared with other animals.[12]
Many cephalopods are social creatures; when isolated from their own kind, some species have been observed shoaling with fish.[13]
Some cephalopods are able to fly through the air for distances of up to 50 m. While cephalopods are not particularly aerodynamic, they achieve these impressive ranges by jet-propulsion; water continues to be expelled from the funnel while the organism is in the air.[14] The animals spread their fins and tentacles to form wings and actively control lift force with body posture.[15] One species, Todarodes pacificus, has been observed spreading tentacles in a flat fan shape with a mucus film between the individual tentacles[15][16] while another, Sepioteuthis sepioidea, has been observed putting the tentacles in a circular arrangement.[17]
Senses
Cephalopods have advanced vision, can detect gravity with statocysts, and have a variety of chemical sense organs.[7]:34 Octopuses use their arms to explore their environment and can use them for depth perception.[7]
Vision
.jpg)
Most cephalopods rely on vision to detect predators and prey, and to communicate with one another.[18] Consequently, cephalopod vision is acute: training experiments have shown that the common octopus can distinguish the brightness, size, shape, and horizontal or vertical orientation of objects. The morphological construction gives cephalopod eyes the same performance as sharks'; however, their construction differs, as cephalopods lack a cornea, and have an everted retina.[18] Cephalopods' eyes are also sensitive to the plane of polarization of light.[19] Unlike many other cephalopods, nautiluses do not have good vision; their eye structure is highly developed, but lacks a solid lens. They have a simple "pinhole" eye through which water can pass. Instead of vision, the animal is thought to use olfaction as the primary sense for foraging, as well as locating or identifying potential mates.
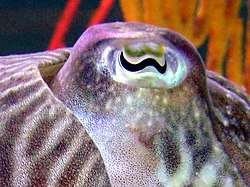
Surprisingly, given their ability to change color, all octopodes[20] and most cephalopods[21][22] are considered to be color blind. Coleoid cephalopods (octopus, squid, cuttlefish) have a single photoreceptor type and lack the ability to determine color by comparing detected photon intensity across multiple spectral channels. When camouflaging themselves, they use their chromatophores to change brightness and pattern according to the background they see, but their ability to match the specific color of a background may come from cells such as iridophores and leucophores that reflect light from the environment.[23] They also produce visual pigments throughout their body, and may sense light levels directly from their body.[24] Evidence of color vision has been found in the sparkling enope squid (Watasenia scintillans),[21][25] which achieves color vision by the use of three distinct retinal molecules: A1, most sensitive to green-blue (484 nm); A2, to blue-green (500 nm), and A4, to blue (470 nm), which bind to its opsin.[26]
In 2015, a novel mechanism for spectral discrimination in cephalopods was described. This relies on the exploitation of chromatic aberration (wavelength-dependence of focal length). Numerical modeling shows that chromatic aberration can yield useful chromatic information through the dependence of image acuity on accommodation. The unusual off-axis slit and annular pupil shapes in cephalopods enhance this ability.[27]
Photoreception
In 2015, molecular evidence was published indicating that cephalopod chromatophores are photosensitive; reverse transcription polymerase chain reactions (RT-PCR) revealed transcripts encoding rhodopsin and retinochrome within the retinas and skin of the longfin inshore squid (Doryteuthis pealeii), and the common cuttlefish (Sepia officinalis) and broadclub cuttlefish (Sepia latimanus). The authors claim this is the first evidence that cephalopod dermal tissues may possess the required combination of molecules to respond to light.[28]
Hearing
Some squids have been shown to detect sound using their statocysts.[29]
Use of light
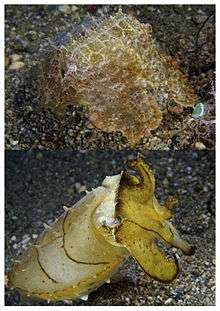
Most cephalopods possess an assemblage of skin components that interact with light. These may include iridophores, leucophores, chromatophores and (in some species) photophores. Chromatophores are colored pigment cells that expand and contract in accordance to produce color and pattern which they can use in a startling array of fashions.[7][28] As well as providing camouflage with their background, some cephalopods bioluminesce, shining light downwards to disguise their shadows from any predators that may lurk below.[7] The bioluminescence is produced by bacterial symbionts; the host cephalopod is able to detect the light produced by these organisms.[30] Bioluminescence may also be used to entice prey, and some species use colorful displays to impress mates, startle predators, or even communicate with one another.[7]
Coloration
Cephalopods can change their colors and patterns in milliseconds, whether for signalling (both within the species and for warning) or active camouflage,[7] as their chromatophores are expanded or contracted.[31] Although color changes appear to rely primarily on vision input, there is evidence that skin cells, specifically chromatophores, can detect light and adjust to light conditions independently of the eyes.[32] Cephalopods can use chromatophores like a muscle, which is why they can change their skin hue as rapidly as they do. Coloration is typically stronger in near-shore species than those living in the open ocean, whose functions tend to be restricted to disruptive camouflage.[7]:2 Most octopuses mimic select structures in their field of view rather than becoming a composite color of their full background.[33]
Evidence of original coloration has been detected in cephalopod fossils dating as far back as the Silurian; these orthoconic individuals bore concentric stripes, which are thought to have served as camouflage.[34] Devonian cephalopods bear more complex color patterns, of unknown function.[35]
Ink
With the exception of the Nautilidae and the species of octopus belonging to the suborder Cirrina,[36] all known cephalopods have an ink sac, which can be used to expel a cloud of dark ink to confuse predators.[20] This sac is a muscular bag which originated as an extension of the hindgut. It lies beneath the gut and opens into the anus, into which its contents – almost pure melanin – can be squirted; its proximity to the base of the funnel means the ink can be distributed by ejected water as the cephalopod uses its jet propulsion.[20] The ejected cloud of melanin is usually mixed, upon expulsion, with mucus, produced elsewhere in the mantle, and therefore forms a thick cloud, resulting in visual (and possibly chemosensory) impairment of the predator, like a smokescreen. However, a more sophisticated behavior has been observed, in which the cephalopod releases a cloud, with a greater mucus content, that approximately resembles the cephalopod that released it (this decoy is referred to as a Pseudomorph). This strategy often results in the predator attacking the pseudomorph, rather than its rapidly departing prey.[20] For more information, see Inking behaviors.
The ink sac of cephalopods has led to a common name of "inkfish",[37] formerly the pen-and-ink fish.[38]
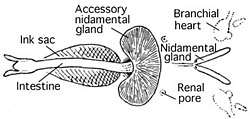
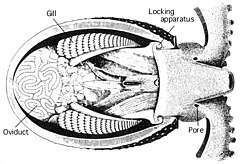
Circulatory system
Cephalopods are the only mollusks with a closed circulatory system. Coleoids have two gill hearts (also known as branchial hearts) that move blood through the capillaries of the gills. A single systemic heart then pumps the oxygenated blood through the rest of the body.[39]
Like most molluscs, cephalopods use hemocyanin, a copper-containing protein, rather than hemoglobin, to transport oxygen. As a result, their blood is colorless when deoxygenated and turns blue when exposed to air.[40]
Respiration
Cephalopods exchange gases with the seawater by forcing water through their gills, which are attached to the roof of the organism.[41]:488[42] Water enters the mantle cavity on the outside of the gills, and the entrance of the mantle cavity closes. When the mantle contracts, water is forced through the gills, which lie between the mantle cavity and the funnel. The water's expulsion through the funnel can be used to power jet propulsion. The gills, which are much more efficient than those of other mollusks, are attached to the ventral surface of the mantle cavity.[42] There is a trade-off with gill size regarding lifestyle. To achieve fast speeds, gills need to be small – water will be passed through them quickly when energy is needed, compensating for their small size. However, organisms which spend most of their time moving slowly along the bottom do not naturally pass much water through their cavity for locomotion; thus they have larger gills, along with complex systems to ensure that water is constantly washing through their gills, even when the organism is stationary.[41] The water flow is controlled by contractions of the radial and circular mantle cavity muscles.[43]
The gills of cephalopods are supported by a skeleton of robust fibrous proteins; the lack of mucopolysaccharides distinguishes this matrix from cartilage.[44][45] The gills are also thought to be involved in excretion, with NH4+ being swapped with K+ from the seawater.[42]
Locomotion and buoyancy

While most cephalopods can move by jet propulsion, this is a very energy-consuming way to travel compared to the tail propulsion used by fish.[46] The efficiency of a propellor-driven waterjet (i.e. Froude efficiency) is greater than a rocket.[47] The relative efficiency of jet propulsion decreases further as animal size increases; paralarvae are far more efficient than juvenile and adult individuals.[48] Since the Paleozoic era, as competition with fish produced an environment where efficient motion was crucial to survival, jet propulsion has taken a back role, with fins and tentacles used to maintain a steady velocity.[4] Whilst jet propulsion is never the sole mode of locomotion,[4]:208 the stop-start motion provided by the jets continues to be useful for providing bursts of high speed – not least when capturing prey or avoiding predators.[4] Indeed, it makes cephalopods the fastest marine invertebrates,[7]:Preface and they can out-accelerate most fish.[41] The jet is supplemented with fin motion; in the squid, the fins flap each time that a jet is released, amplifying the thrust; they are then extended between jets (presumably to avoid sinking).[48] Oxygenated water is taken into the mantle cavity to the gills and through muscular contraction of this cavity, the spent water is expelled through the hyponome, created by a fold in the mantle. The size difference between the posterior and anterior ends of this organ control the speed of the jet the organism can produce.[49] The velocity of the organism can be accurately predicted for a given mass and morphology of animal.[50] Motion of the cephalopods is usually backward as water is forced out anteriorly through the hyponome, but direction can be controlled somewhat by pointing it in different directions.[51] Some cephalopods accompany this expulsion of water with a gunshot-like popping noise, thought to function to frighten away potential predators.[52]
Cephalopods employ a similar method of propulsion despite their increasing size (as they grow) changing the dynamics of the water in which they find themselves. Thus their paralarvae do not extensively use their fins (which are less efficient at low Reynolds numbers) and primarily use their jets to propel themselves upwards, whereas large adult cephalopods tend to swim less efficiently and with more reliance on their fins.[48]
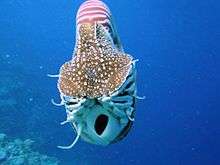
Early cephalopods are thought to have produced jets by drawing their body into their shells, as Nautilus does today.[53] Nautilus is also capable of creating a jet by undulations of its funnel; this slower flow of water is more suited to the extraction of oxygen from the water.[53] The jet velocity in Nautilus is much slower than in coleoids, but less musculature and energy is involved in its production.[54] Jet thrust in cephalopods is controlled primarily by the maximum diameter of the funnel orifice (or, perhaps, the average diameter of the funnel)[55]:440 and the diameter of the mantle cavity.[56] Changes in the size of the orifice are used most at intermediate velocities.[55] The absolute velocity achieved is limited by the cephalopod's requirement to inhale water for expulsion; this intake limits the maximum velocity to eight body-lengths per second, a speed which most cephalopods can attain after two funnel-blows.[55] Water refills the cavity by entering not only through the orifices, but also through the funnel.[55] Squid can expel up to 94% of the fluid within their cavity in a single jet thrust.[47] To accommodate the rapid changes in water intake and expulsion, the orifices are highly flexible and can change their size by a factor of twenty; the funnel radius, conversely, changes only by a factor of around 1.5.[55]
Some octopus species are also able to walk along the seabed. Squids and cuttlefish can move short distances in any direction by rippling of a flap of muscle around the mantle.
While most cephalopods float (i.e. are neutrally buoyant or nearly so; in fact most cephalopods are about 2–3% denser than seawater[13]), they achieve this in different ways.[46] Some, such as Nautilus, allow gas to diffuse into the gap between the mantle and the shell; others allow purer water to ooze from their kidneys, forcing out denser salt water from the body cavity;[46] others, like some fish, accumulate oils in the liver;[46] and some octopuses have a gelatinous body with lighter chlorine ions replacing sulfate in the body chemistry.[46]
The Macrotritopus defilippi, or the sand-dwelling octopus, was seen mimicking both the coloration and the swimming movements of the sand-dwelling flounder Bothus lunatus to avoid predators. The octopuses were able to flatten their bodies and put their arms back to appear the same as the flounders as well as move with the same speed and movements.[57]
Females of two species, Ocythoe tuberculata and Haliphron atlanticus, have evolved a true swim bladder.[58]
Shell
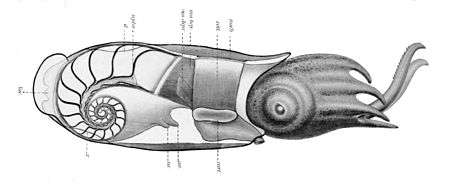
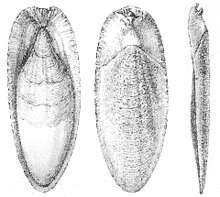
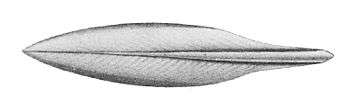
Nautiluses are the only extant cephalopods with a true external shell. However, all molluscan shells are formed from the ectoderm (outer layer of the embryo); in cuttlefish (Sepia spp.), for example, an invagination of the ectoderm forms during the embryonic period, resulting in a shell (cuttlebone) that is internal in the adult.[59] The same is true of the chitinous gladius of squid[59] and octopuses.[60] Cirrate octopods have arch-shaped cartilaginous fin supports,[61] which are sometimes referred to as a "shell vestige" or "gladius".[62] The Incirrina have either a pair of rod-shaped stylets or no vestige of an internal shell,[63] and some squid also lack a gladius.[64] The shelled coleoids do not form a clade or even a paraphyletic group.[65] The Spirula shell begins as an organic structure, and is then very rapidly mineralized.[66] Shells that are "lost" may be lost by resorption of the calcium carbonate component.[67]
Females of the octopus genus Argonauta secrete a specialized paper-thin egg case in which they reside, and this is popularly regarded as a "shell", although it is not attached to the body of the animal and has a separate evolutionary origin.
The largest group of shelled cephalopods, the ammonites, are extinct, but their shells are very common as fossils.
The deposition of carbonate, leading to a mineralized shell, appears to be related to the acidity of the organic shell matrix (see Mollusc shell); shell-forming cephalopods have an acidic matrix, whereas the gladius of squid has a basic matrix.[68] The basic arrangement of the cephalopod outer wall is: an outer (spherulitic) prismatic layer, a laminar (nacreous) layer and an inner prismatic layer. The thickness of every layer depends on the taxa.[69] In modern cephalopods, the Ca carbonate is aragonite. As for other mollusc shells or coral skeletons, the smallest visible units are irregular rounded granules.[70]
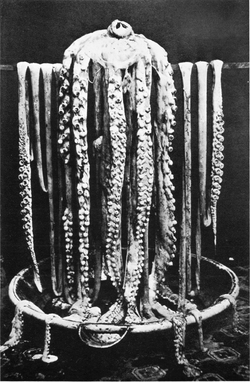
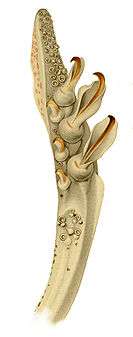
Right: Detail of the tentacular club of Abraliopsis morisi
Head appendages
Cephalopods, as the name implies, have muscular appendages extending from their heads and surrounding their mouths. These are used in feeding, mobility, and even reproduction. In coleoids they number eight or ten. Decapods such as cuttlefish and squid have five pairs. The longer two, termed tentacles, are actively involved in capturing prey;[1]:225 they can lengthen rapidly (in as little as 15 milliseconds[1]:225). In giant squid they may reach a length of 8 metres. They may terminate in a broadened, sucker-coated club.[1]:225 The shorter four pairs are termed arms, and are involved in holding and manipulating the captured organism.[1]:225 They too have suckers, on the side closest to the mouth; these help to hold onto the prey.[1]:226 Octopods only have four pairs of sucker-coated arms, as the name suggests, though developmental abnormalities can modify the number of arms expressed.[71]
The tentacle consists of a thick central nerve cord (which must be thick to allow each sucker to be controlled independently)[72] surrounded by circular and radial muscles. Because the volume of the tentacle remains constant, contracting the circular muscles decreases the radius and permits the rapid increase in length. Typically a 70% lengthening is achieved by decreasing the width by 23%.[1]:227 The shorter arms lack this capability.
The size of the tentacle is related to the size of the buccal cavity; larger, stronger tentacles can hold prey as small bites are taken from it; with more numerous, smaller tentacles, prey is swallowed whole, so the mouth cavity must be larger.[73]
Externally shelled nautilids (Nautilus and Allonautilus) have on the order of 90 finger-like appendages, termed tentacles, which lack suckers but are sticky instead, and are partly retractable.
Feeding
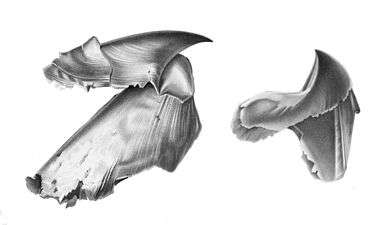
All living cephalopods have a two-part beak;[7]:7 most have a radula, although it is reduced in most octopus and absent altogether in Spirula.[7]:7[74]:110 They feed by capturing prey with their tentacles, drawing it into their mouth and taking bites from it.[20] They have a mixture of toxic digestive juices, some of which are manufactured by symbiotic algae, which they eject from their salivary glands onto their captured prey held in their mouths. These juices separate the flesh of their prey from the bone or shell.[20] The salivary gland has a small tooth at its end which can be poked into an organism to digest it from within.[20]
The digestive gland itself is rather short.[20] It has four elements, with food passing through the crop, stomach and caecum before entering the intestine. Most digestion, as well as the absorption of nutrients, occurs in the digestive gland, sometimes called the liver. Nutrients and waste materials are exchanged between the gut and the digestive gland through a pair of connections linking the gland to the junction of the stomach and caecum.[20] Cells in the digestive gland directly release pigmented excretory chemicals into the lumen of the gut, which are then bound with mucus passed through the anus as long dark strings, ejected with the aid of exhaled water from the funnel.[20] Cephalopods tend to concentrate ingested heavy metals in their body tissue.[75]
Radula

The cephalopod radula consists of multiple symmetrical rows of up to nine teeth[76] – thirteen in fossil classes.[77] The organ is reduced or even vestigial in certain octopus species and is absent in Spirula.[77] The teeth may be homodont (i.e. similar in form across a row), heterodont (otherwise), or ctenodont (comb-like).[77] Their height, width and number of cusps is variable between species.[77] The pattern of teeth repeats, but each row may not be identical to the last; in the octopus, for instance, the sequence repeats every five rows.[77]:79
Cephalopod radulae are known from fossil deposits dating back to the Ordovician.[78] They are usually preserved within the cephalopod's body chamber, commonly in conjunction with the mandibles; but this need not always be the case;[79] many radulae are preserved in a range of settings in the Mason Creek.[80] Radulae are usually difficult to detect, even when they are preserved in fossils, as the rock must weather and crack in exactly the right fashion to expose them; for instance, radulae have only been found in nine of the 43 ammonite genera,[81] and they are rarer still in non-ammonoid forms: only three pre-Mesozoic species possess one.[78]
Excretory system
Most cephalopods possess a single pair of large nephridia. Filtered nitrogenous waste is produced in the pericardial cavity of the branchial hearts, each of which is connected to a nephridium by a narrow canal. The canal delivers the excreta to a bladder-like renal sac, and also resorbs excess water from the filtrate. Several outgrowths of the lateral vena cava project into the renal sac, continuously inflating and deflating as the branchial hearts beat. This action helps to pump the secreted waste into the sacs, to be released into the mantle cavity through a pore.[82]
Nautilus, unusually, possesses four nephridia, none of which are connected to the pericardial cavities.
The incorporation of ammonia is important for shell formation in terrestrial molluscs and other non-molluscan lineages.[83] Because protein (i.e. flesh) is a major constituent of the cephalopod diet, large amounts of ammonium ions are produced as waste. The main organs involved with the release of this excess ammonium are the gills.[84] The rate of release is lowest in the shelled cephalopods Nautilus and Sepia as a result of their using nitrogen to fill their shells with gas to increase buoyancy.[84] Other cephalopods use ammonium in a similar way, storing the ions (as ammonium chloride) to reduce their overall density and increase buoyancy.[84]
Reproduction and life cycle
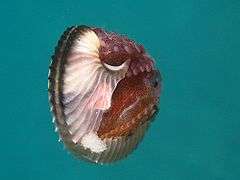
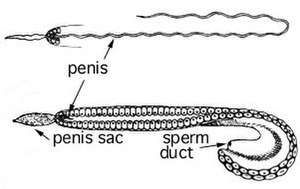
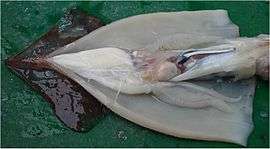
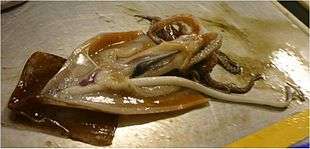
Cephalopods are a diverse group of species, but share common life history traits, for example, they have a rapid growth rate and short life spans.[85] Stearns (1992) suggested that in order to produce the largest possible number of viable offspring, spawning events depend on the ecological environmental factors of the organism. The majority of cephalopods do not provide parental care to their offspring, except, for example, octopus, which helps this organism increase the survival rate of their offspring.[85] Marine species' life cycles are affected by various environmental conditions.[86] The development of a cephalopod embryo can be greatly affected by temperature, oxygen saturation, pollution, light intensity, and salinity.[85] These factors are important to the rate of embryonic development and the success of hatching of the embryos. Food availability also plays an important role in the reproductive cycle of cephalopods. A limitation of food influences the timing of spawning along with their function and growth.[86] Spawning time and spawning vary among marine species; it's correlated with temperature, though cephalopods in shallow water spawn in cold months so that the offspring would hatch at warmer temperatures. Breeding can last from several days to a month.[85]
Sexual maturity
Cephalopods that are sexually mature and of adult size begin spawning and reproducing. After the transfer of genetic material to the following generation, the adult cephalopods then die.[85] Sexual maturation in male and female cephalopods can be observed internally by the enlargement of gonads and accessory glands.[87] Mating would be a poor indicator of sexual maturation in females; they can receive sperm when not fully reproductively mature and store them until they are ready to fertilize the eggs.[86] Males are more aggressive in their pre-mating competition when in the presence of immature females than when competing for a sexually mature female.[88] Most cephalopod males develop a hectocotylus, an arm tip which is capable of transferring their spermatozoa into the female mantel cavity. Though not all species use a hectocotylus; for example, the adult nautilus releases a spadix.[89] An indication of sexual maturity of females is the development of brachial photophores to attract mates.[90]
Fertilization
Cephalopods are not broadcast spawners. During the process of fertilization, the females use sperm provided by the male via external fertilization. Internal fertilization is seen only in octopodes.[87] The initiation of copulation begins when the male catches a female and wraps his arm around her, either in a "male to female neck" position or mouth to mouth position, depending on the species. The males then initiate the process of fertilization by contracting their mantle several times to release the spermatozoa.[91] Cephalopods often mate several times, which influences males to mate longer with females that have previously, nearly tripling the number of contractions of the mantle.[91] To ensure the fertilization of the eggs, female cephalopods release a sperm-attracting peptide through the gelatinous layers of the egg to direct the spermatozoa. Female cephalopods lay eggs in clutches; each egg is composed of a protective coat to ensure the safety of the developing embryo when released into the water column. Reproductive strategies differ between cephalopod species. In giant Pacific octopus, large eggs are laid in a den; it will often take several days to lay all of them.[87] Once the eggs are released and attached to a sheltered substrate, the females then die,[87] making them semelparous. In some species of cephalopods, egg clutches are anchored to substrates by a mucilaginous adhesive substance. These eggs are swelled with perivitelline fluid (PVF), a hypertonic fluid that prevents premature hatching.[92] Fertilized egg clusters are neutrally buoyant depending on the depth that they were laid, but can also be found in substrates such as sand, a matrix of corals, or seaweed.[86] Because these species do not provide parental care for their offspring, egg capsules can be injected with ink by the female in order to camouflage the embryos from predators.[86]
Male–male competition
Most cephalopods engage in aggressive sex: a protein in the male capsule sheath stimulates this behavior. They also engage in male–male aggression, where larger males tend to win the interactions.[85] When a female is near, the males charge one another continuously and flail their arms. If neither male backs away, the arms extend to the back, exposing the mouth, followed by the biting of arm tips.[93] During mate competition males also participate in a technique called flushing. This technique is used by the second male attempting to mate with a female. Flushing removes spermatophores in the buccal cavity that was placed there by the first mate by forcing water into the cavity.[85] Another behavior that males engage in is sneaker mating or mimicry – smaller males adjust their behavior to that of a female in order to reduce aggression. By using this technique, they are able to fertilize the eggs while the larger male is distracted by a different male.[93] During this process, the sneaker males quickly insert drop-like sperm into the seminal receptacle.[94]
Mate choice
Mate choice is seen in cuttlefish species, where females prefer some males over others, though characteristics of the preferred males are unknown.[85] A hypothesis states that females reject males by olfactory cues rather than visual cues.[85] Several cephalopod species are polyandrous- accepting and storing multiple male spermatophores, which has been identified by DNA fingerprinting.[91] Females are no longer receptive to mating attempts when holding their eggs in their arms. Females can store sperm in two places (1) the buccal cavity where recently mated males place their spermatophores, and (2) the internal sperm-storage receptacles where sperm packages from previous males are stored.[85] Spermatophore storage results in sperm competition; which states that the female controls which mate fertilizes the eggs. In order to reduce this sort of competition, males develop agonistic behaviors like mate guarding and flushing.[85] The Hapalochlaena lunulata, or the blue-ringed octopus, readily mates with both males and females.[95]
Sexual dimorphism
In a variety of marine organisms, it is seen that females are larger in size compared to the males in some closely related species. In some lineages, such as the blanket octopus, males become structurally smaller and smaller resembling a term, "dwarfism" dwarf males usually occurs at low densities.[96] The blanket octopus male is an example of sexual-evolutionary dwarfism; females grow 10,000 to 40,000 times larger than the males and the sex ratio between males and females can be distinguished right after hatching of the eggs.[96]
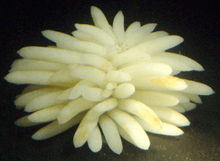
Embryology
Cephalopod eggs span a large range of sizes, from 1 to 30 mm in diameter.[97] The fertilised ovum initially divides to produce a disc of germinal cells at one pole, with the yolk remaining at the opposite pole. The germinal disc grows to envelop and eventually absorb the yolk, forming the embryo. The tentacles and arms first appear at the hind part of the body, where the foot would be in other molluscs, and only later migrate towards the head.[82][98]
The funnel of cephalopods develops on the top of their head, whereas the mouth develops on the opposite surface.[99]:86 The early embryological stages are reminiscent of ancestral gastropods and extant Monoplacophora.[98]
The shells develop from the ectoderm as an organic framework which is subsequently mineralized.[59] In Sepia, which has an internal shell, the ectoderm forms an invagination whose pore is sealed off before this organic framework is deposited.[59]
Development
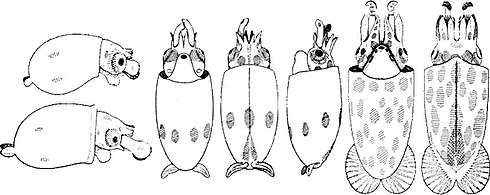
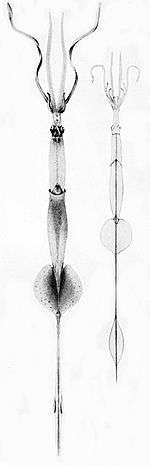
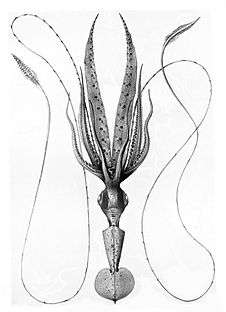
Right: A mature Chiroteuthis veranyi. This species has some of the longest tentacles in proportion to its size of any known cephalopod.
The length of time before hatching is highly variable; smaller eggs in warmer waters are the fastest to hatch, and newborns can emerge after as little as a few days. Larger eggs in colder waters can develop for over a year before hatching.[97]
The process from spawning to hatching follows a similar trajectory in all species, the main variable being the amount of yolk available to the young and when it is absorbed by the embryo.[97]
Unlike most other mollusks, cephalopods do not have a morphologically distinct larval stage. Instead, the juveniles are known as paralarvae. They quickly learn how to hunt, using encounters with prey to refine their strategies.[97]
Growth in juveniles is usually allometric, whilst adult growth is isometric.[100]
Evolution
The traditional view of cephalopod evolution holds that they evolved in the Late Cambrian from a monoplacophoran-like ancestor[101] with a curved, tapering shell,[102] which was closely related to the gastropods (snails).[103] The similarity of the early shelled cephalopod Plectronoceras to some gastropods was used in support of this view. The development of a siphuncle would have allowed the shells of these early forms to become gas-filled (thus buoyant) in order to support them and keep the shells upright while the animal crawled along the floor, and separated the true cephalopods from putative ancestors such as Knightoconus, which lacked a siphuncle.[103] Neutral or positive buoyancy (i.e. the ability to float) would have come later, followed by swimming in the Plectronocerida and eventually jet propulsion in more derived cephalopods.[104]
However, some morphological evidence is difficult to reconcile with this view, and the redescription of Nectocaris pteryx, which did not have a shell and appeared to possess jet propulsion in the manner of "derived" cephalopods, complicated the question of the order in which cephalopod features developed – provided Nectocaris is a cephalopod at all.[105]
Early cephalopods were likely predators near the top of the food chain.[20] After the late Cambrian extinction led to the disappearance of many Anomalocaridids, predatory niches became available for other animals.[106] During the Ordovician period the primitive cephalopods underwent pulses of diversification[107] to become diverse and dominant in the Paleozoic and Mesozoic seas.[108]
In the Early Palaeozoic, their range was far more restricted than today; they were mainly constrained to sublittoral regions of shallow shelves of the low latitudes, and usually occurred in association with thrombolites.[109] A more pelagic habit was gradually adopted as the Ordovician progressed.[109] Deep-water cephalopods, whilst rare, have been found in the Lower Ordovician – but only in high-latitude waters.[109] The mid-Ordovician saw the first cephalopods with septa strong enough to cope with the pressures associated with deeper water, and could inhabit depths greater than 100–200 m.[107] The direction of shell coiling would prove to be crucial to the future success of the lineages; endogastric coiling would only permit large size to be attained with a straight shell, whereas exogastric coiling – initially rather rare – permitted the spirals familiar from the fossil record to develop, with their corresponding large size and diversity.[110] (Endogastric mean the shell is curved so as the ventral or lower side is longitudinally concave (belly in); exogastric means the shell is curved so as the ventral side is longitudinally convex (belly out) allowing the funnel to be pointed backward beneath the shell.)[110]
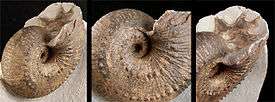
The ancestors of coleoids (including most modern cephalopods) and the ancestors of the modern nautilus, had diverged by the Floian Age of the Early Ordovician Period, over 470 million years ago.[109][111] The Bactritida, a Silurian–Triassic group of orthocones, are widely held to be paraphyletic to the coleoids and ammonoids, that is, the latter groups arose from within the Bactritida.[112]:393 An increase in the diversity of the coleoids and ammonoids is observed around the start of the Devonian period and corresponds with a profound increase in fish diversity. This could represent the origin of the two derived groups.[112]
Unlike most modern cephalopods, most ancient varieties had protective shells. These shells at first were conical but later developed into curved nautiloid shapes seen in modern nautilus species. Competitive pressure from fish is thought to have forced the shelled forms into deeper water, which provided an evolutionary pressure towards shell loss and gave rise to the modern coleoids, a change which led to greater metabolic costs associated with the loss of buoyancy, but which allowed them to recolonize shallow waters.[103]:36 However, some of the straight-shelled nautiloids evolved into belemnites, out of which some evolved into squid and cuttlefish. The loss of the shell may also have resulted from evolutionary pressure to increase maneuverability, resulting in a more fish-like habit.[1]:289
There has been debate on the embryological origin of cephalopod appendages.[113] Until the mid-twentieth century, the "Arms as Head" hypothesis was widely recognized. In this theory, the arms and tentacles of cephalopods look similar to the head appendages of gastropods, suggesting that they might be homologous structures. Cephalopod appendages surround the mouth, so logically they could be derived from embryonic head tissues.[114] However, the "Arms as Foot" hypothesis, proposed by Adolf Naef in 1928, has increasingly been favoured;[113] for example, fate mapping of limb buds in the chambered nautilus indicates that limb buds originate from "foot" embryonic tissues.[115]
Genetics
The sequencing of a full Cephalopod genome has remained challenging to researchers due to the length and repetition of their DNA.[116] The characteristics of Cephalopod genomes were initially hypothesized to be the result of entire genome duplications. Following the full sequencing of a California two-spot octopus, the genome showed similar patterns to other marine invertebrates with significant additions to the genome assumed to be unique to Cephalopods. No evidence of full genome duplication was found.[117]
Within the California two-spot octopus genome there are substantial replications of two gene families. Significantly, the expanded gene families were only previously known to exhibit replicative behaviour within vertebrates.[117] The first gene family was identified as the Protocadherins which are attributed to neuron development. Protocadherins function as cell adhesion molecules, essential for synaptic specificity. The mechanism for Protocadherin gene family replication in vertebrates is attributed to complex splicing, or cutting and pasting, from a locus. Following the sequencing of the California two-spot octopus, researchers found that the Prorocadherin gene family in Cephalopods has expanded in the genome due to tandem gene duplication. The different replication mechanisms for Protocadherin genes indicate an independent evolution of Protocadherin gene expansion in vertebrates and invertebrates.[117] Analysis of individual Cephalopod Protocadherin genes indicate independent evolution between species of Cephalopod. A species of shore squid Doryteuthis pealeii with expanded Protocadherin gene families differ significantly from those of the California two-spot octopus suggesting gene expansion did not occur before speciation within Cephalopods. Despite different mechanisms for gene expansion, the two-spot octopus Protocadherin genes were more similar to vertebrates than squid, suggesting a convergent evolution mechanism. The second gene family known as C2H2 are small proteins that function as zinc transcription factors. C2H2 are understood to moderate DNA, RNA and protein functions within the cell.[116]
The sequenced California two spot octopus genome also showed a significant presence of transposable elements as well as transposon expression. Although the role of transposable elements in marine vertebrates is still relatively unknown, significant expression of transposons in nervous system tissues have been observed.[118] In a study conducted on vertebrates, the expression of transposons during development in Drosophila melanogaster activated genomic diversity between neurons.[119] This diversity has been linked to increased memory and learning in mammals. The connection between transposons and increased neuron capability may provide insight into the observed intelligence, memory and function of Cephalopods.[118]
Phylogeny
The approximate consensus of extant cephalopod phylogeny, after Strugnell et al. 2007, is shown in the cladogram.[65] Mineralized taxa are in bold. The attachment of the clade including Sepia and Spirula is unclear; either of the points marked with an asterisk may represent the root of this clade.
Cephalopoda |
| |||||||||||||||||||||||||||||||||||||||||||||||||||||||||
The internal phylogeny of the cephalopods is difficult to constrain; many molecular techniques have been adopted, but the results produced are conflicting.[65][120] Nautilus tends to be considered an outgroup, with Vampyroteuthis forming an outgroup to other squid; however in one analysis the nautiloids, octopus and teuthids plot as a polytomy.[65] Some molecular phylogenies do not recover the mineralized coleoids (Spirula, Sepia, and Metasepia) as a clade; however, others do recover this more parsimonious-seeming clade, with Spirula as a sister group to Sepia and Metasepia in a clade that had probably diverged before the end of the Triassic.[121][122]
Molecular estimates for clade divergence vary. One 'statistically robust' estimate has Nautilus diverging from Octopus at 415 ± 24 million years ago.[123]
Taxonomy
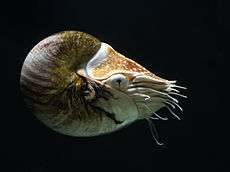
.jpg)
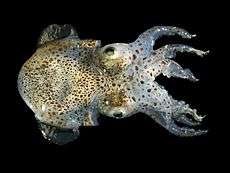

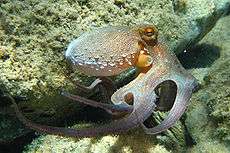
The classification presented here, for recent cephalopods, follows largely from Current Classification of Recent Cephalopoda (May 2001), for fossil cephalopods takes from Arkell et al. 1957, Teichert and Moore 1964, Teichert 1988, and others. The three subclasses are traditional, corresponding to the three orders of cephalopods recognized by Bather.[124]
Class Cephalopoda († indicates extinct groups)
- Subclass Nautiloidea: Fundamental ectocochliate cephalopods that provided the source for the Ammonoidea and Coleoidea.
- Order † Plectronocerida: the ancestral cephalopods from the Cambrian Period
- Order † Ellesmerocerida (500 to 470 Ma)
- Order † Endocerida (485 to 430 Ma)
- Order † Actinocerida (480 to 312 Ma)
- Order † Discosorida (482 to 392 Ma)
- Order † Pseudorthocerida (432 to 272 Ma)
- Order † Tarphycerida (485 to 386 Ma)
- Order † Oncocerida (478.5 to 324 Ma)
- Order Nautilida (extant; 410.5 Ma to present)
- Order † Orthocerida (482.5 to 211.5 Ma)
- Order † Ascocerida (478 to 412 Ma)
- Order † Bactritida (418.1 to 260.5 Ma)
- Subclass † Ammonoidea: Ammonites (479 to 66 Ma)
- Order † Goniatitida (388.5 to 252 Ma)
- Order † Ceratitida (254 to 200 Ma)
- Order † Ammonitida (215 to 66 Ma)
- Subclass Coleoidea (410.0 Ma-Rec)
- Cohort † Belemnoidea: Belemnites and kin
- Genus † Jeletzkya
- Order † Aulacocerida (265 to 183 Ma)
- Order † Phragmoteuthida (189.6 to 183 Ma)
- Order † Hematitida (339.4 to 318.1 Ma)
- Order † Belemnitida (339.4 to 66 Ma)
- Genus † Belemnoteuthis (189.6 to 183 Ma)
- Cohort Neocoleoidea
- Superorder Decapodiformes (also known as Decabrachia or Decembranchiata)
- Superorder Octopodiformes (also known as Vampyropoda)
- Family † Trachyteuthididae
- Order Vampyromorphida: Vampire squid
- Order Octopoda: octopus
- Superorder † Palaeoteuthomorpha
- Order † Boletzkyida
- Cohort † Belemnoidea: Belemnites and kin
Other classifications differ, primarily in how the various decapod orders are related, and whether they should be orders or families.
Suprafamilial classification of the Treatise
This is the older classification that combines those found in parts K and L of the Treatise on Invertebrate Paleontology, which forms the basis for and is retained in large part by classifications that have come later.
Nautiloids in general (Teichert and Moore, 1964) sequence as given.
- Subclass † Endoceratoidea. Not used by Flower, e.g. Flower and Kummel 1950, interjocerids included in the Endocerida.
- Order † Endocerida
- Order † Intejocerida
- Subclass † Actinoceratoidea Not used by Flower, ibid
- Order † Actinocerida
- Subclass Nautiloidea Nautiloidea in the restricted sense.
- Order † Ellesmerocerida Plectronocerida subsequently split off as separate order.
- Order † Orthocerida Includes orthocerids and pseudorthocerids
- Order † Ascocerida
- Order † Oncocerida
- Order † Discosorida
- Order † Tarphycerida
- Order † Barrandeocerida A polyphyletic group now included in the Tarphycerida
- Order Nautilida
- Subclass † Bactritoidea
- Order † Bactritida
Paleozoic Ammonoidea (Miller, Furnish and Schindewolf, 1957)
- Suborder † Anarcestina
- Suborder † Clymeniina
- Suborder † Goniatitina
- Suborder † Prolecanitina
Mesozoic Ammonoidea (Arkel et al., 1957)
- Suborder † Ceratitina
- Suborder † Phylloceratina
- Suborder † Lytoceratina
- Suborder † Ammonitina
Subsequent revisions include the establishment of three Upper Cambrian orders, the Plectronocerida, Protactinocerida, and Yanhecerida; separation of the pseudorthocerids as the Pseudorthocerida, and elevating orthoceratid as the Subclass Orthoceratoidea.
Shevyrev classification
Shevyrev (2005) suggested a division into eight subclasses, mostly comprising the more diverse and numerous fossil forms,[125][126] although this classification has been criticized as arbitrary.[127]
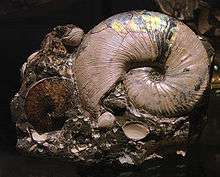
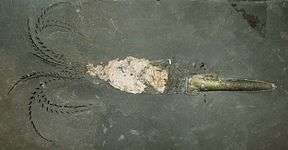
Class Cephalopoda
- Subclass † Ellesmeroceratoidea
- Order † Plectronocerida (501 to 490 Ma)
- Order † Protactinocerida
- Order † Yanhecerida
- Order † Ellesmerocerida (500 to 470 Ma)
- Subclass † Endoceratoidea (485 to 430 Ma)
- Order † Endocerida (485 to 430 Ma)
- Order † Intejocerida (485 to 480 Ma)
- Subclass † Actinoceratoidea
- Order † Actinocerida (480 to 312 Ma)
- Subclass Nautiloidea (490.0 Ma- Rec)
- Order † Basslerocerida (490 to 480 Ma)
- Order † Tarphycerida (485 to 386 Ma)
- Order † Lituitida (485 to 480 Ma)
- Order † Discosorida (482 to 392 Ma)
- Order † Oncocerida (478.5 to 324 Ma)
- Order Nautilida (410.5 Ma-Rec)
- Subclass † Orthoceratoidea (482.5 to 211.5 Ma)
- Order † Orthocerida (482.5 to 211.5 Ma)
- Order † Ascocerida (478 to 412 Ma)
- Order † Dissidocerida (479 to 457.5 Ma)
- Order † Bajkalocerida
- Subclass † Bactritoidea (422 to 252 Ma)
- Subclass † Ammonoidea (410 to 66 Ma)
- Subclass Coleoidea (410.0 Ma-rec)[128]
Cladistic classification
Another recent system divides all cephalopods into two clades. One includes nautilus and most fossil nautiloids. The other clade (Neocephalopoda or Angusteradulata) is closer to modern coleoids, and includes belemnoids, ammonoids, and many orthocerid families. There are also stem group cephalopods of the traditional Ellesmerocerida that belong to neither clade.[129][130]
The coleoids, despite some doubts,[1]:289 appear from molecular data to be monophyletic.[131]
In culture

Ancient seafaring people were aware of cephalopods, as evidenced by artworks such as a stone carving found in the archaeological recovery from Bronze Age Minoan Crete at Knossos (1900 – 1100 BC) has a depiction of a fisherman carrying an octopus.[132] The terrifyingly powerful Gorgon of Greek mythology may have been inspired by the octopus or squid, the octopus's body representing the severed head of Medusa, the beak as the protruding tongue and fangs, and its tentacles as the snakes.[133]
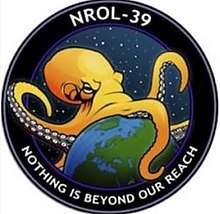
The Kraken are legendary sea monsters of giant proportions said to dwell off the coasts of Norway and Greenland, usually portrayed in art as giant cephalopods attacking ships. Linnaeus included it in the first edition of his 1735 Systema Naturae.[134][135] A Hawaiian creation myth says that the present cosmos is the last of a series which arose in stages from the ruins of the previous universe. In this account, the octopus is the lone survivor of the previous, alien universe.[136] The Akkorokamui is a gigantic tentacled monster from Ainu folklore.[137]
A battle with an octopus plays a significant role in Victor Hugo's book Travailleurs de la mer (Toilers of the Sea), relating to his time in exile on Guernsey.[138] Ian Fleming's 1966 short story collection Octopussy and The Living Daylights, and the 1983 James Bond film were partly inspired by Hugo's book.[139]
Japanese erotic art, shunga, includes ukiyo-e woodblock prints such as Katsushika Hokusai's 1814 print Tako to ama (The Dream of the Fisherman's Wife), in which an ama diver is sexually intertwined with a large and a small octopus.[140][141] The print is a forerunner of tentacle erotica.[142] The biologist P. Z. Myers noted in his science blog, Pharyngula, that octopuses appear in "extraordinary" graphic illustrations involving women, tentacles, and bare breasts.[143][144]
Since it has numerous arms emanating from a common center, the octopus is often used as a symbol for a powerful and manipulative organization, usually negatively.[145]
See also
- Cephalopod size
- Cephalopod eye
- Cephalopod intelligence
- Pain in cephalopods
- Kraken
- List of nautiloids
- List of ammonites
References
- Wilbur, Karl M.; Trueman, E.R.; Clarke, M.R., eds. (1985), The Mollusca, 11. Form and Function, New York: Academic Press, ISBN 0-12-728702-7
- Queiroz, K.; Cantino, P.D.; Gauthier, J.A. (2020). Phylonyms: A Companion to the PhyloCode. CRC Press. p. 1843. ISBN 978-1-138-33293-5.
- "Welcome to CephBase". CephBase. Retrieved 29 January 2016.
- Wilbur, Karl M.; Clarke, M.R.; Trueman, E.R., eds. (1985), The Mollusca, 12. Paleontology and neontology of Cephalopods, New York: Academic Press, ISBN 0-12-728702-7
- Bartol, I. K.; Mann, R.; Vecchione, M. (2002). "Distribution of the euryhaline squid Lolliguncula brevis in Chesapeake Bay: effects of selected abiotic factors". Marine Ecology Progress Series. 226: 235–247. Bibcode:2002MEPS..226..235B. doi:10.3354/meps226235.
- "Are there any freshwater cephalopods?". ABC Science. 16 January 2013.
- Nixon, Marion; Young, J. Z. (2003). The Brains and Lives of Cephalopods. New York: Oxford University Press. ISBN 978-0-19-852761-9.
- Tricarico, E.; Amodio, P.; Ponte, G.; Fiorito, G. (2014). "Cognition and recognition in the cephalopod mollusc Octopus vulgaris: coordinating interaction with environment and conspecifics". In Witzany, G. (ed.). Biocommunication of Animals. Springer. pp. 337–349. ISBN 978-94-007-7413-1.
- Budelmann, B. U. (1995). "The cephalopod nervous system: What evolution has made of the molluscan design". In Breidbach, O.; Kutsch, W. (eds.). The nervous systems of invertebrates: An evolutionary and comparative approach. ISBN 978-3-7643-5076-5.
- Chung, Wen-Sung; Kurniawan, Nyoman D.; Marshall, N. Justin (2020). "Toward an MRI-Based Mesoscale Connectome of the Squid Brain". iScience. 23 (1): 100816. doi:10.1016/j.isci.2019.100816. PMC 6974791. PMID 31972515.
- Raven, Peter et al. (2003). Biology, p. 669. McGraw-Hill Education, New York. ISBN 9780073383071.
- Tasaki, I.; Takenaka, T. (1963). "Resting and action potential of squid giant axons intracellularly perfused with sodium-rich solutions". Proceedings of the National Academy of Sciences of the United States of America. 50 (4): 619–626. Bibcode:1963PNAS...50..619T. doi:10.1073/pnas.50.4.619. PMC 221236. PMID 14077488.
- Packard, A. (1972). "Cephalopods and fish: the limits of convergence". Biological Reviews. 47 (2): 241–307. doi:10.1111/j.1469-185X.1972.tb00975.x.
- Macia, Silvia; Robinson, Michael P.; Craze, Paul; Dalton, Robert; Thomas, James D. (2004). "New observations on airborne jet propulsion (flight) in squid, with a review of previous reports". Journal of Molluscan Studies. 70 (3): 297–299. doi:10.1093/mollus/70.3.297.
- Muramatsu, K.; Yamamoto, J.; Abe, T.; Sekiguchi, K.; Hoshi, N.; Sakurai, Y. (2013). "Oceanic squid do fly". Marine Biology. 160 (5): 1171–1175. doi:10.1007/s00227-013-2169-9.
- "Scientists Unravel Mystery of Flying Squid". Ocean Views. National Geographic. 20 February 2013.
- Jabr, Ferris (2 August 2010). "Fact or Fiction: Can a Squid Fly out of Water?". Scientific American.
- Serb, J. M.; Eernisse, D. J. (2008). "Charting Evolution's Trajectory: Using Molluscan Eye Diversity to Understand Parallel and Convergent Evolution". Evolution: Education and Outreach. 1 (4): 439–447. doi:10.1007/s12052-008-0084-1.
- Wells, Martin J. (2011). "Part M, Chapter 4: Physiology of Coleoids". Treatise Online. Lawrence, Kansas, USA. doi:10.17161/to.v0i0.4226. Archived from the original on 2016-08-22. Retrieved 2013-05-10.(subscription required)
- Boyle, Peter; Rodhouse, Paul (2004). Cephalopods : ecology and fisheries. Blackwell. doi:10.1002/9780470995310.ch2. ISBN 978-0-632-06048-1.
- Messenger, John B.; Hanlon, Roger T. (1998). Cephalopod Behaviour. Cambridge: Cambridge University Press. pp. 17–21. ISBN 978-0-521-64583-6.
- Chung, Wen-Sung; Marshall, N. Justin (2016-09-14). "Comparative visual ecology of cephalopods from different habitats". Proceedings of the Royal Society B: Biological Sciences. 283 (1838): 20161346. doi:10.1098/rspb.2016.1346. ISSN 0962-8452. PMC 5031660. PMID 27629028.
- Hanlon and Messenger, 68.
- Mäthger, L.; Roberts, S.; Hanlon, R. (2010). "Evidence for distributed light sensing in the skin of cuttlefish, Sepia officinalis". Biology Letters. 6 (5): 600–603. doi:10.1098/rsbl.2010.0223. PMC 2936158. PMID 20392722.
- Michinomae, M.; Masuda, H.; Seidou, M.; Kito, Y. (1994). "Structural basis for wavelength discrimination in the banked retina of the firefly squid Watasenia scintillans". Journal of Experimental Biology. 193 (1): 1–12. PMID 9317205.
- Seidou, M.; Sugahara, M.; Uchiyama, H.; Hiraki, K.; Hamanaka, T.; Michinomae, M.; Yoshihara, K.; Kito, Y. (1990). "On the three visual pigments in the retina of the firefly squid, Watasenia scintillans". Journal of Comparative Physiology A. 166 (6). doi:10.1007/BF00187321.
- Stubbs, A. L.; Stubbs, C. W. (2015). "A novel mechanism for color vision: Pupil shape and chromatic aberration can provide spectral discrimination for 'color blind' organisms". bioRxiv 10.1101/017756.
- Kingston, A. C.; Kuzirian, A. M.; Hanlon, R. T.; Cronin, T. W. (2015). "Visual phototransduction components in cephalopod chromatophores suggest dermal photoreception". Journal of Experimental Biology. 218 (10): 1596–1602. doi:10.1242/jeb.117945. PMID 25994635.
- "The cephalopods can hear you". BBC News. 2009-06-15. Retrieved 2010-04-28.
- Tong, D.; Rozas, S.; Oakley, H.; Mitchell, J.; Colley, J.; Mcfall-Ngai, J. (Jun 2009). "Evidence for light perception in a bioluminescent organ". Proceedings of the National Academy of Sciences of the United States of America. 106 (24): 9836–9841. Bibcode:2009PNAS..106.9836T. doi:10.1073/pnas.0904571106. ISSN 0027-8424. PMC 2700988. PMID 19509343.
- "integument (mollusks)."Encyclopædia Britannica. 2009. Encyclopædia Britannica 2006 Ultimate Reference Suite DVD
- Ramirez, M. D.; Oakley, T. H (2015). "Eye-independent, light-activated chromatophore expansion (LACE) and expression of phototransduction genes in the skin of Octopus bimaculoides" (PDF). Journal of Experimental Biology. 218 (10): 1513–1520. doi:10.1242/jeb.110908. PMC 4448664. PMID 25994633.
- Josef, Noam; Amodio, Piero; Fiorito, Graziano; Shashar, Nadav (2012-05-23). "Camouflaging in a Complex Environment—Octopuses Use Specific Features of Their Surroundings for Background Matching". PLOS ONE. 7 (5): e37579. Bibcode:2012PLoSO...737579J. doi:10.1371/journal.pone.0037579. ISSN 1932-6203. PMC 3359305. PMID 22649542.
- Manda, Štěpán; Turek, Vojtěch (2009). "Minute Silurian oncocerid nautiloids with unusual color patterns". Acta Palaeontologica Polonica. 54 (3): 503–512. doi:10.4202/app.2008.0062.
- Turek, Vojtěch (2009). "Colour patterns in Early Devonian cephalopods from the Barrandian Area: Taphonomy and taxonomy". Acta Palaeontologica Polonica. 54 (3): 491–502. doi:10.4202/app.2007.0064.
- Hanlon, Roger T.; Messenger, John B. (1999). Cephalopod Behaviour. Cambridge University Press. p. 2. ISBN 978-0-521-64583-6.
- "inkfish". Merriam-Webster. Retrieved 1 February 2018.
- Bickerdyke, John (1895). Sea Fishing. London: Longmans, Green, and Co. p. 114.
the common squid or calamary (Loligo vulgaris). It is sometimes called the pen-and-ink fish, on account of its ink bag, and the delicate elongated shell which is found within it.
- Wells, M.J. (1 April 1980). "Nervous control of the heartbeat in octopus". Journal of Experimental Biology. 85 (1): 111–28. PMID 7373208.
- Ghiretti-Magaldi, A. (October 1992). "The Pre-history of Hemocyanin. The Discovery of Copper in the Blood of Molluscs". Cellular and Molecular Life Sciences. 48 (10): 971–972. doi:10.1007/BF01919143.
- Gilbert, Daniel L.; Adelman, William J.; Arnold, John M. (1990). Squid as Experimental Animals (illustrated ed.). Springer. ISBN 978-0-306-43513-3.
- Schipp, Rudolf; Mollenhauer, Stephan; Boletzky, Sigurd (1979). "Electron Microscopical and Histochemical Studies of Differentiation and Function of the Cephalopod Gill (Sepia Officinalis L.)". Zoomorphologie. 93 (3): 193–207. doi:10.1007/BF00993999.
- Bone, Q.; Brown, E. R.; Travers, G. (1994). "On the respiratory flow in the cuttlefish Sepia Officinalis" (PDF). Journal of Experimental Biology. 194 (1): 153–165. PMID 9317534.
- Cole, A.; Hall, B. (2009). "Cartilage differentiation in cephalopod molluscs". Zoology. 112 (1): 2–15. doi:10.1016/j.zool.2008.01.003. PMID 18722759.
- See also http://tolweb.org/articles/?article_id=4200
- Wilbur, Karl M.; Clarke, M.R.; Trueman, E.R., eds. (1985), "11: Evolution of Buoyancy and Locomotion in recent cephalopods", The Mollusca, 12. Paleontology and neontology of Cephalopods, New York: Academic Press, ISBN 0-12-728702-7
- Anderson, E.; Demont, M. (2000). "The mechanics of locomotion in the squid Loligo pealei: Locomotory function and unsteady hydrodynamics of the jet and intramantle pressure". Journal of Experimental Biology. 203 (18): 2851–2863. PMID 10952883.
- Bartol, I. K.; Krueger, P. S.; Thompson, J. T.; Stewart, W. J. (2008). "Swimming dynamics and propulsive efficiency of squids throughout ontogeny". Integrative and Comparative Biology. 48 (6): 720–733. doi:10.1093/icb/icn043. PMID 21669828.
- Shea, E.; Vecchione, M. (2002). "Quantification of ontogenetic discontinuities in three species of oegopsid squids using model II piecewise linear regression". Marine Biology. 140 (5): 971–979. doi:10.1007/s00227-001-0772-7.
- Johnson, W.; Soden, P. D.; Trueman, E. R. (February 1972). "A study in jet propulsion: an analysis of the motion of the squid, Loligo vulgaris". Journal of Experimental Biology. 56 (1972): 155–165.
- Campbell, Reece & Mitchell (1999), p. 612.
- Guerra, A.; Martinell, X.; González, A. F.; Vecchione, M.; Gracia, J.; Martinell, J. (2007). "A new noise detected in the ocean". Journal of the Marine Biological Association of the United Kingdom. 87 (5): 1255–1256. doi:10.1017/S0025315407058225. hdl:10261/27009.
- Wells, Martin J.; O'Dor, R. K. (July 1991). "Jet Propulsion and the Evolution of the Cephalopods". Bulletin of Marine Science. 49 (1): 419–432(14).
- Chamberlain, J., Jr. (1993). "Locomotion in ancient seas: Constraint and opportunity in cephalopod adaptive design". Geobios. 26 (Suppl. 1): 49–61. doi:10.1016/S0016-6995(06)80360-8.
- O'Dor, R. K. (1988). "The forces acting on swimming squid". Journal of Experimental Biology. 137: 421–442.
- O'Dor, R. K.; Hoar, J. A. (2000). "Does geometry limit squid growth?". ICES Journal of Marine Science. 57: 8–14. doi:10.1006/jmsc.1999.0502.
- Hanlon, Roger T.; Watson, Anya C.; Barbosa, Alexandra (2010-02-01). "A 'Mimic Octopus' in the Atlantic: Flatfish Mimicry and Camouflage by Macrotritopus defilippi". The Biological Bulletin. 218 (1): 15–24. doi:10.1086/BBLv218n1p15. hdl:1912/4811. ISSN 0006-3185. PMID 20203250.
- "The argonaut shell: Gas-mediated buoyancy control in a pelagic octopus".
- Baratte, S.; Andouche, A.; Bonnaud, L. (2007). "Engrailed in cephalopods: a key gene related to the emergence of morphological novelties". Development Genes and Evolution. 217 (5): 353–362. doi:10.1007/s00427-007-0147-2. PMID 17394016.
- von Boletzky, S. (2004). "'Ammonoïdes nus': un défi pour la phylogénie des céphalopodes ?" ['Nude ammonoids': a challenge to cephalopod phylogeny?]. Geobios. 37: 117–118. doi:10.1016/j.geobios.2003.01.009.
- Gibson, R. N.; Atkinson, R. J. A.; Gordon, J. D. M., eds. (2006). Oceanography and Marine Biology: An Annual Review. CRC Press. p. 288. ISBN 978-1420006391.
- Aldred, R. G.; Nixon, M.; Young, J. Z. (1983). "Cirrothauma murrayi Chun, a finned octopod". Philosophical Transactions of the Royal Society B: Biological Sciences. 301 (1103): 1–54. Bibcode:1983RSPTB.301....1A. doi:10.1098/rstb.1983.0021.
- Fuchs, D.; Ifrim, C.; Stinnesbeck, W. (2008). "A new Palaeoctopus (Cephalopoda: Coleoidea) from the Late Cretaceous of Vallecillo, north-eastern Mexico, and implications for the evolution of Octopoda". Palaeontology. 51 (5): 1129–1139. doi:10.1111/j.1475-4983.2008.00797.x.
- von Boletzky, Sigurd (July 1991). "The terminal spine of sepiolid hatchlings: its development and functional morphology (Mollusca, Cephalopoda)". Bulletin of Marine Science. 49: 107–112.
- Strugnell, J.; Nishiguchi, M. K. (2007). "Molecular phylogeny of coleoid cephalopods (Mollusca: Cephalopoda) inferred from three mitochondrial and six nuclear loci: a comparison of alignment, implied alignment and analysis methods". Journal of Molluscan Studies. 73 (4): 399–410. doi:10.1093/mollus/eym038.
- Warnke, K.; Keupp, H. (2005). "Spirula – a window to the embryonic development of ammonoids? Morphological and molecular indications for a palaeontological hypothesis". Facies. 51 (1–4): 60–65. doi:10.1007/s10347-005-0054-9.
- Furuhashi, T.; Schwarzinger, C.; Miksik, I.; Smrz, M.; Beran, A. (2009). "Molluscan shell evolution with review of shell calcification hypothesis". Comparative Biochemistry and Physiology B. 154 (3): 351–371. doi:10.1016/j.cbpb.2009.07.011. PMID 19665573.
- Dauphin, Y. (1996). "The organic matrix of coleoid cephalopod shells: molecular weights and isoelectric properties of the soluble matrix in relation to biomineralization processes". Marine Biology. 125 (3): 525–529. doi:10.1007/BF00353265 (inactive 2020-06-05).
- Dauphin, Y. (1983). Les subdivisions majeures de la classe des céphalopodes : bases de la systématique actuelle : apport de l'analyse microstructurale. These Doct. Etat, Université Paris Sud. OCLC 972899981.
- Dauphin, Y. (2001). "Nanostructures de la nacre des tests de céphalopodes actuels". Paläontologische Zeitschrift. 75 (1): 113–122. doi:10.1007/bf03022601. ISSN 0031-0220.
- Toll, R. B.; Binger, L. C. (1991). "Arm anomalies: Cases of supernumerary development and bilateral agenesis of arm pairs in Octopoda (Mollusca, Cephalopoda)". Zoomorphology. 110 (6): 313–316. doi:10.1007/BF01668021.
- Anatomy of the Common Squid. 1912.
- Nixon 1988 in Wippich, M. G. E.; Lehmann, J. (2004). "Allocrioceras from the Cenomanian (mid-Cretaceous) of the Lebanon and its bearing on the palaeobiological interpretation of heteromorphic ammonites". Palaeontology. 47 (5): 1093–1107. doi:10.1111/j.0031-0239.2004.00408.x.
- Wilbur, Karl M.; Clarke, M.R.; Trueman, E.R., eds. (1985), "5", The Mollusca, 12. Paleontology and neontology of Cephalopods, New York: Academic Press, ISBN 0-12-728702-7
- C.Michael Hogan. 2011. Celtic Sea. eds. P.Saundry & C.Cleveland. Encyclopedia of Earth. National Council for Science and the Environment. Washington DC.
- "Cephalopod radula". Tree of Life web project.
- Nixon, M. (1995). "A nomenclature for the radula of the Cephalopoda (Mollusca) – living and fossil". Journal of Zoology. 236: 73–81. doi:10.1111/j.1469-7998.1995.tb01785.x.
- Gabbott, S. E. (1999). "Orthoconic cephalopods and associated fauna from the late Ordovician Soom Shale Lagerstatte, South Africa". Palaeontology. 42: 123–148. doi:10.1111/1475-4983.00065.
- Landman, Neil H.; Davis, Richard Arnold; Mapes, Royal H., eds. (2007). Cephalopods present and past: new insights and fresh perspectives. Springer. ISBN 978-1-4020-6461-6.
- Richardson & ... (1977). Fossils of the Mason Creek.
- Kruta, I.; Landman, N.; Rouget, I.; Cecca, F.; Tafforeau, P. (2011). "The role of ammonites in the Mesozoic marine food web revealed by jaw preservation". Science. 331 (6013): 70–72. Bibcode:2011Sci...331...70K. doi:10.1126/science.1198793. PMID 21212354.
- Barnes, Robert D. (1982). Invertebrate Zoology. Philadelphia, PA: Holt-Saunders International. pp. 450–460. ISBN 978-0-03-056747-6.
- Loest, R. A. (1979). "Ammonia Volatilization and Absorption by Terrestrial Gastropods_ a Comparison between Shelled and Shell-Less Species". Physiological Zoology. 52 (4): 461–469. doi:10.1086/physzool.52.4.30155937. JSTOR 30155937.
- Boucher-Rodoni, R.; Mangold, K. (1994). "Ammonia production in cephalopods, physiological and evolutionary aspects". Marine and Freshwater Behaviour and Physiology. 25 (1–3): 53–60. doi:10.1080/10236249409378907.
- Vidal, Erica A. G. Advances in Cephalopod Science: Biology, Ecology, Cultivation and Fisheries.
- Rodrigues, M.; Guerra; Troncoso (2010). "The embryonic phase and its implication in the hatchling size and condition of Atlantic bobtail squid Sepiola Atlantica". Helgoland Marine Research. 65 (2): 211–216. Bibcode:2011HMR....65..211R. doi:10.1007/s10152-010-0217-0.
- Arkhipkin, A. I. (1992). "Reproductive system structure, development and function in cephalopods with a new general scale for maturity stages". Journal of Northwest Atlantic Fishery Science. 12: 63–74. doi:10.2960/j.v12.a7.
- Mohanty, Sobhi; Ojanguren, Alfredo F.; Fuiman, Lee A. (2014-07-01). "Aggressive male mating behavior depends on female maturity in Octopus bimaculoides". Marine Biology. 161 (7): 1521–1530. doi:10.1007/s00227-014-2437-3. ISSN 0025-3162.
- Saunders, W. B; Spinosa, C. (1978). "Sexual dimorphism in Nautilus from Palau". Paleobiology. 4 (3): 349–358. doi:10.1017/S0094837300006047.
- Young, R. B. (1975). "A Systematic Approach to Planning Occupational Programs". Community College Review. 3 (2): 19–25. doi:10.1177/009155217500300204.
- Squires, Z. E; Norman, M. D; Stuart-Fox, D. (2013). "Mating behaviour and general spawning patterns of the southern dumpling squid Euprymna tasmanica". Journal of Molluscan Studies. 79 (3): 263–269. doi:10.1093/mollus/eyt025.
- Marthy, H. J.; Hauser, R; Scholl, A. (1976). "Natural tranquilizer in cephalopod eggs". Nature. 261 (5560): 496–7. Bibcode:1976Natur.261..496M. doi:10.1038/261496a0. PMID 945466.
- Norman, M. D.; Lu, C. C. (1997). "Redescription of the southern dumpling squid Euprymna tasmanica and a revision of the genus Euprymna (Cephalopoda: Sepiolidae)". Journal of the Marine Biological Association of the United Kingdom. 77 (4): 1109–1137. doi:10.1017/s0025315400038662.
- Iwata, Y.; Ito, K.; Sakurai, Y. (2008). "Effect of low temperature on mating behavior of squid Loligo bleekeri". Fisheries Science. 74 (6): 1345–1347. doi:10.1111/j.1444-2906.2008.01664.x.
- Cheng, Mary W.; Caldwell, Roy L. (July 2000). "Sex identification and mating in the blue-ringed octopus, Hapalochlaena lunulata". Animal Behaviour. 60 (1): 27–33. doi:10.1006/anbe.2000.1447. ISSN 0003-3472. PMID 10924200. S2CID 32899443.
- Fairbairn, D. (2013). "Blanket Octopus: Drifting Females and Dwarf Males". Odd couples: Extraordinary differences between the sexes in the animal kingdom. Princeton University Press. pp. 104–115.
- Von Boletzky, S. (2003). Biology of early life stages in cephalopod molluscs. Advances in Marine Biology. 44. pp. 143–203. doi:10.1016/S0065-2881(03)44003-0. ISBN 978-0-12-026144-4. PMID 12846042.
- Shigeno, S.; Sasaki, T.; Moritaki, T.; Kasugai, T.; Vecchione, M.; Agata, K. (Jan 2008). "Evolution of the cephalopod head complex by assembly of multiple molluscan body parts: Evidence from Nautilus embryonic development". Journal of Morphology. 269 (1): 1–17. doi:10.1002/jmor.10564. PMID 17654542.
- Gilbert, Daniel L.; Adelman, William J.; Arnold, John M. (1990). Squid as experimental animals. New York: Plenum Press. ISBN 978-0-306-43513-3.
- Moltschaniwskyj, Natalie A. (2004). "Understanding the process of growth in cephalopods". Marine and Freshwater Research. 55 (4): 379–386. doi:10.1071/MF03147.
- Lemche, H.; Wingstrand, K. G. (1959). "The anatomy of Neopilina galatheae Lemche, 1957 (Mollusca, Tryblidiacea)" (Link to free full text + plates). Galathea Report. 3: 9–73.
- Wingstrand, K. G. (1985). "On the anatomy and relationships of Recent Monoplacophora". Galathea Report. 16: 7–94. Archived from the original (Link to free full text + plates) on 2016-03-03. Retrieved 2009-03-23.
- Boyle, P.; Rodhouse, P. (2005). "Origin and Evolution". Cephalopods. p. 36. doi:10.1002/9780470995310.ch3. ISBN 9780470995310.
- Kröger, B. R. (2007). "Some Lesser Known Features of the Ancient Cephalopod Order Ellesmerocerida (Nautiloidea, Cephalopoda)". Palaeontology. 50 (3): 565–572. doi:10.1111/j.1475-4983.2007.00644.x.
- Smith, Martin R.; Caron, Jean-Bernard (2010). "Primitive soft-bodied cephalopods from the Cambrian". Nature. 465 (7297): 427–428. Bibcode:2010Natur.465..427B. doi:10.1038/465427a. PMID 20505713.
- Jain, Sreepat (2016). Fundamentals of Invertebrate Palaeontology: Macrofossils. Springer. p. 73. ISBN 978-81-322-3658-0.
- Kröger, B.; Yun-bai, Y. B. (2009). "Pulsed cephalopod diversification during the Ordovician". Palaeogeography, Palaeoclimatology, Palaeoecology. 273 (1–2): 174–201. Bibcode:2009PPP...273..174K. doi:10.1016/j.palaeo.2008.12.015.
- Dzik, J. (1981). "Origin of the Cephalopoda" (PDF). Acta Palaeontologica Polonica. 26 (2): 161–191.
- Kröger, B. R.; Servais, T.; Zhang, Y.; Kosnik, M. (2009). "The origin and initial rise of pelagic cephalopods in the Ordovician". PLOS ONE. 4 (9): e7262. Bibcode:2009PLoSO...4.7262K. doi:10.1371/journal.pone.0007262. PMC 2749442. PMID 19789709.
- Holland, C. H. (1987). "The nautiloid cephalopods: a strange success: President's anniversary address 1986". Journal of the Geological Society. 144 (1): 1–15. Bibcode:1987JGSoc.144....1H. doi:10.1144/gsjgs.144.1.0001.
- Kröger, Björn (2006). "Early growth-stages and classification of orthoceridan cephalopods of the Darriwillian (Middle Ordovician) of Baltoscandia". Lethaia. 39 (2): 129–139. doi:10.1080/00241160600623749.
- Young, R. E.; Vecchione, M.; Donovan, D. T. (1998). "The evolution of coleoid cephalopods and their present biodiversity and ecology". South African Journal of Marine Science. 20 (1): 393–420. doi:10.2989/025776198784126287.
- Tanabe, K. (2008). Cephalopods – Present and Past. Tokyo: Tokai University Press.
- Basil, Jennifer; Bahctinova, Irina; Kuroiwa, Kristine; Lee, Nandi; Mims, Desiree; Preis, Michael; Soucier, Christian (2005-09-01). "The function of the rhinophore and the tentacles of Nautilus pompilius L. (Cephalopoda, Nautiloidea) in orientation to odor". Marine and Freshwater Behaviour and Physiology. 38 (3): 209–221. doi:10.1080/10236240500310096.
- Shigeno, Shuichi; Sasaki, Takenori; Moritaki, Takeya; Kasugai, Takashi; Vecchione, Michael; Agata, Kiyokazu (January 2008). "Evolution of the cephalopod head complex by assembly of multiple molluscan body parts: Evidence from Nautilus embryonic development". Journal of Morphology. 269 (1): 1–17. doi:10.1002/jmor.10564. PMID 17654542.
- O’Brien, Caitlin E.; Roumbedakis, Katina; Winkelmann, Inger E. (2018-06-06). "The Current State of Cephalopod Science and Perspectives on the Most Critical Challenges Ahead From Three Early-Career Researchers". Frontiers in Physiology. 9: 700. doi:10.3389/fphys.2018.00700. ISSN 1664-042X. PMC 6014164. PMID 29962956.
- Albertin, Caroline B.; Simakov, Oleg; Mitros, Therese; Wang, Z. Yan; Pungor, Judit R.; Edsinger-Gonzales, Eric; Brenner, Sydney; Ragsdale, Clifton W.; Rokhsar, Daniel S. (August 2015). "The octopus genome and the evolution of cephalopod neural and morphological novelties". Nature. 524 (7564): 220–224. Bibcode:2015Natur.524..220A. doi:10.1038/nature14668. ISSN 0028-0836. PMC 4795812. PMID 26268193.
- Gehring, Mary A. (2013-02-04), Plant Transposons and Genome Dynamics in Evolution, Wiley-Blackwell, pp. 117–142, doi:10.1002/9781118500156.ch7, ISBN 978-1-118-50015-6 Missing or empty
|title=
(help);|chapter=
ignored (help) - Erwin, Jennifer A.; Marchetto, Maria C.; Gage, Fred H. (August 2014). "Mobile DNA elements in the generation of diversity and complexity in the brain". Nature Reviews Neuroscience. 15 (8): 497–506. doi:10.1038/nrn3730. ISSN 1471-003X. PMC 4443810. PMID 25005482.
- Strugnell, J.; Norman, M.; Jackson, J.; Drummond, A.; Cooper, A. (2005). "Molecular phylogeny of coleoid cephalopods (Mollusca: Cephalopoda) using a multigene approach; the effect of data partitioning on resolving phylogenies in a Bayesian framework". Molecular Phylogenetics and Evolution. 37 (2): 426–441. doi:10.1016/j.ympev.2005.03.020. PMID 15935706.
- Strugnell, J.; Jackson, J.; Drummond, A. J.; Cooper, A. (2006). "Divergence time estimates for major cephalopod groups: evidence from multiple genes". Cladistics. 22: 89–96. doi:10.1111/j.1096-0031.2006.00086.x.
- Carlini, D. B.; Reece, K. S.; Graves, J. E. (2000). "Actin gene family evolution and the phylogeny of coleoid cephalopods (Mollusca: Cephalopoda)". Molecular Biology and Evolution. 17 (9): 1353–1370. doi:10.1093/oxfordjournals.molbev.a026419. PMID 10958852.
- Bergmann, S.; Lieb, B.; Ruth, P.; Markl, J. (2006). "The hemocyanin from a living fossil, the cephalopod Nautilus pompilius: protein structure, gene organization, and evolution". Journal of Molecular Evolution. 62 (3): 362–374. Bibcode:2006JMolE..62..362B. doi:10.1007/s00239-005-0160-x. PMID 16501879.
- Bather, F.A. (1888b). "Professor Blake and Shell-Growth in Cephalopoda". Annals and Magazine of Natural History. 6. 1 (6): 421–426. doi:10.1080/00222938809460761.
- Shevyrev, A. A. (2005). "The Cephalopod Macrosystem: A Historical Review, the Present State of Knowledge, and Unsolved Problems: 1. Major Features and Overall Classification of Cephalopod Mollusks". Paleontological Journal. 39 (6): 606–614. Translated from Paleontologicheskii Zhurnal No. 6, 2005, 33–42.
- Shevyrev, A. A. (2006). "The cephalopod macrosystem; a historical review, the present state of knowledge, and unsolved problems; 2, Classification of nautiloid cephalopods". Paleontological Journal. 40 (1): 46–54. doi:10.1134/S0031030106010059.
- Kroger, B. "Peer review in the Russian 'Paleontological Journal'". Archived from the original on 2009-08-31.
- Bather, F.A. (1888a). "Shell-growth in Cephalopoda (Siphonopoda)". Annals and Magazine of Natural History. 6. 1 (4): 298–310. doi:10.1080/00222938809460727.
- Berthold, Thomas; Engeser, Theo (1987). "Phylogenetic analysis and systematization of the Cephalopoda (Mollusca)". Verhandlungen Naturwissenschaftlichen Vereins in Hamburg. 29: 187–220.
- Engeser, Theo (1997). "Fossil Nautiloidea Page". Archived from the original on 2006-09-25.
- Lindgren, A. R.; Giribet, G.; Nishiguchi, M. K. (2004). "A combined approach to the phylogeny of Cephalopoda (Mollusca)". Cladistics. 20 (5): 454–486. CiteSeerX 10.1.1.693.2026. doi:10.1111/j.1096-0031.2004.00032.x.
- Hogan, C. Michael (22 December 2007). "Knossos fieldnotes". The Modern Antiquarian.
- Wilk, Stephen R. (2000). Medusa: Solving the Mystery of the Gorgon. Oxford University Press. ISBN 978-0-19-988773-6.
- "Caroli Linnaei Systema naturae sistens regna tria naturae". google.com.
- Smedley, Edward; Rose, Hugh James; Rose, Henry John (1845). Encyclopaedia Metropolitana, Or, Universal Dictionary of Knowledge: Comprising the Twofold Advantage of a Philosophical and an Alphabetical Arrangement, with Appropriate Engravings. B. Fellowes. pp. 255–.
- Dixon, Roland Burrage (1916). Oceanic. The Mythology of All Races. 9. Marshall Jones Company. pp. 2–.
- Batchelor, John (1901). The Ainu and Their Folklore. London: The Religious Tract Society.
- Chisholm, Hugh, ed. (1911). Encyclopædia Britannica (11th ed.). Cambridge University Press. .
- Cohen-Vrignaud, Gerard (2012). "On Octopussies, or the Anatomy of Female Power". Differences. 23 (2): 32–61. doi:10.1215/10407391-1533520.
- Fritze, Sointu; Suojoki, Saara (2000). Forbidden Images: Erotic Art from Japan's Edo Period (in Finnish). Helsingin kaupungin taidemuseo. pp. 23–28. ISBN 978-951-8965-54-4.
- Uhlenbeck, Chris; Margarita Winkel; Ellis Tinios; Amy Reigle Newland (2005). Japanese Erotic Fantasies: Sexual Imagery of the Edo Period. Hotei. p. 161. ISBN 978-90-74822-66-4.
- Briel, Holger (2010). Berninger, Mark; Ecke, Jochen; Haberkorn, Gideon (eds.). The Roving Eye Meets Traveling Pictures: The Field of Vision and the Global Rise of Adult Manga. Comics As a Nexus of Cultures: Essays on the Interplay of Media, Disciplines. McFarland. p. 203. ISBN 978-0-7864-3987-4.
- Myers, P. Z. (17 May 2017). "Extraordinary Octopus Illustrations". Pharyngula. Retrieved 18 March 2017.
- Myers, P. Z. (29 October 2006). "Definitely not safe for work". Pharyngula. Retrieved 18 March 2017.
- Smith, S. (26 February 2010). "Why Mark Zuckerberg Octopus Cartoon Evokes 'Nazi Propaganda,' German Paper Apologizes". iMediaEthics. Retrieved 31 May 2017.
Further reading
- Barskov, I. S.; Boiko, M. S.; Konovalova, V. A.; Leonova, T. B.; Nikolaeva, S. V. (2008). "Cephalopods in the marine ecosystems of the Paleozoic". Paleontological Journal. 42 (11): 1167–1284. doi:10.1134/S0031030108110014. A comprehensive overview of Paleozoic cephalopods.
- Campbell, Neil A.; Reece, Jane B.; Mitchell, Lawrence G. (1999). Biology, fifth edition. Menlo Park, California: Addison Wesley Longman, Inc. ISBN 978-0-8053-6566-5.CS1 maint: ref=harv (link)
- Felley, J., Vecchione, M., Roper, C. F. E., Sweeney, M. & Christensen, T., 2001–2003: Current Classification of Recent Cephalopoda. National Museum of Natural History: Department of Systematic Biology: Invertebrate Zoology: Cephalopods
- N. Joan Abbott, Roddy Williamson, Linda Maddock. Cephalopod Neurobiology. Oxford University Press, 1995. ISBN 0-19-854790-0
- Marion Nixon & John Z. Young. The brains and lives of Cephalopods. Oxford University Press, 2003. ISBN 0-19-852761-6
- Hanlon, Roger T. & John B. Messenger. Cephalopod Behaviour. Cambridge University Press, 1996. ISBN 0-521-42083-0
- Martin Stevens & Sami Merilaita. Animal camouflage: mechanisms and function. Cambridge University Press, 2011. ISBN 0-521-19911-5
- Rodhouse, P. G.; Nigmatullin, Ch. M. (1996). "Role as Consumers". Philosophical Transactions of the Royal Society B: Biological Sciences. 351 (1343): 1003–1022. doi:10.1098/rstb.1996.0090.
- Classification key to modern cephalopods: ftp://ftp.fao.org/docrep/fao/009/a0150e/a0150e03.pdf%5B%5D
External links
![]() |
Wikispecies has information related to Cephalopoda |
![]() |
The Wikibook Dichotomous Key has a page on the topic of: Cephalopoda |
![]() |
Wikisource has the text of the 1911 Encyclopædia Britannica article Cephalopoda. |
- Fish vs. Cephalopods
- TONMO.COM – The Octopus News Magazine Online – cephalopod articles and discussion
- Scientific American: Can a Squid Fly Out of the Water?
- Roger Hanlon's Seminar: "Rapid Adaptive Camouflage and Signaling in Cephalopods"
- Deep Sea Dwelling Bristle Worms
![]() |
Wikimedia Commons has media related to Cephalopoda. |