Acetyl-CoA carboxylase
Acetyl-CoA carboxylase (ACC) is a biotin-dependent enzyme that catalyzes the irreversible carboxylation of acetyl-CoA to produce malonyl-CoA through its two catalytic activities, biotin carboxylase (BC) and carboxyltransferase (CT). ACC is a multi-subunit enzyme in most prokaryotes and in the chloroplasts of most plants and algae, whereas it is a large, multi-domain enzyme in the endoplasmic reticulum of most eukaryotes. The most important function of ACC is to provide the malonyl-CoA substrate for the biosynthesis of fatty acids.[1] The activity of ACC can be controlled at the transcriptional level as well as by small molecule modulators and covalent modification. The human genome contains the genes for two different ACCs[2]—ACACA[3] and ACACB.[4]
Acetyl-CoA carboxylase | |||||||||
---|---|---|---|---|---|---|---|---|---|
Identifiers | |||||||||
EC number | 6.4.1.2 | ||||||||
CAS number | 9023-93-2 | ||||||||
Databases | |||||||||
IntEnz | IntEnz view | ||||||||
BRENDA | BRENDA entry | ||||||||
ExPASy | NiceZyme view | ||||||||
KEGG | KEGG entry | ||||||||
MetaCyc | metabolic pathway | ||||||||
PRIAM | profile | ||||||||
PDB structures | RCSB PDB PDBe PDBsum | ||||||||
Gene Ontology | AmiGO / QuickGO | ||||||||
|
Acetyl-CoA carboxylase alpha | |
---|---|
Identifiers | |
Symbol | ACACA |
Alt. symbols | ACAC, ACC1, ACCA |
NCBI gene | 31 |
HGNC | 84 |
OMIM | 601557 |
RefSeq | NM_198839 |
UniProt | Q13085 |
Other data | |
EC number | 6.4.1.2 |
Locus | Chr. 17 q21 |
Acetyl-CoA carboxylase beta | |
---|---|
Identifiers | |
Symbol | ACACB |
Alt. symbols | ACC2, ACCB |
NCBI gene | 32 |
HGNC | 85 |
OMIM | 200350 |
RefSeq | NM_001093 |
UniProt | O00763 |
Other data | |
EC number | 6.4.1.2 |
Locus | Chr. 12 q24.1 |
Structure
Prokaryotes and plants have multi-subunit ACCs composed of several polypeptides. Biotin carboxylase (BC) activity, biotin carboxyl carrier protein (BCCP), and carboxyl transferase (CT) activity are each contained on a different subunit. The stoichiometry of these subunits in the ACC holoenzyme differs amongst organisms.[1] Humans and most eukaryotes have evolved an ACC with CT and BC catalytic domains and BCCP domains on a single polypeptide. Most plants also have this homomeric form in cytosol.[5] ACC functional regions, starting from the N-terminus to C-terminus are the biotin carboxylase (BC), biotin binding (BB), carboxyl transferase (CT), and ATP-binding (AB). AB lies within BC. Biotin is covalently attached through an amide bond to the long side chain of a lysine reside in BB. As BB is between BC and CT regions, biotin can easily translocate to both of the active sites where it is required.
In mammals where two isoforms of ACC are expressed, the main structural difference between these isoforms is the extended ACC2 N-terminus containing a mitochondrial targeting sequence.[1]
- Biotin carboxylase subunit of E. coli acetyl-CoA carboxylase
- Biotin carboxyl carrier protein subunit of E. coli acetyl-CoA carboxylase
- Carboxyl transferase subunit of E. coli acetyl-CoA carboxylase
Genes
The polypeptides composing the multi-subunit ACCs of prokaryotes and plants are encoded by distinct genes. In Escherichia coli, accA encodes the alpha subunit of the acetyl-CoA carboxylase,[6] and accD encodes its beta subunit.[7]
Mechanism
The overall reaction of ACAC(A,B) proceeds by a two-step mechanism.[8] The first reaction is carried out by BC and involves the ATP-dependent carboxylation of biotin with bicarbonate serving as the source of CO2. The carboxyl group is transferred from biotin to acetyl CoA to form malonyl CoA in the second reaction, which is catalyzed by CT.
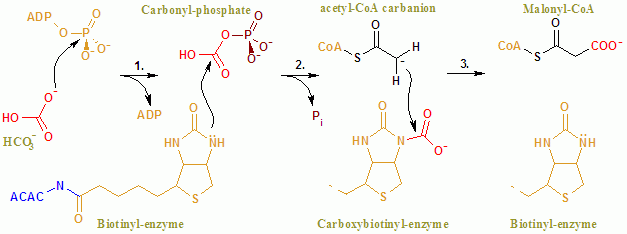
In the active site, the reaction proceeds with extensive interaction of the residues Glu296 and positively charged Arg338 and Arg292 with the substrates.[9] Two Mg2+ are coordinated by the phosphate groups on the ATP, and are required for ATP binding to the enzyme. Bicarbonate is deprotonated by Glu296, although in solution, this proton transfer is unlikely as the pKa of bicarbonate is 10.3. The enzyme apparently manipulates the pKa to facilitate the deprotonation of bicarbonate. The pKa of bicarbonate is decreased by its interaction with positively charged side chains of Arg338 and Arg292. Furthermore, Glu296 interacts with the side chain of Glu211, an interaction that has been shown to cause an increase in the apparent pKa. Following deprotonation of bicarbonate, the oxygen of the bicarbonate acts as a nucleophile and attacks the gamma phosphate on ATP. The carboxyphosphate intermediate quickly decomposes to CO2 and PO43−. The PO43− deprotonates biotin, creating an enolate, stabilized by Arg338, that subsequently attacks CO2 resulting in the production of carboxybiotin.[9] The carboxybiotin translocates to the carboxyl transferase (CT) active site, where the carboxyl group is transferred to acetyl-CoA. In contrast to the BC domain, little is known about the reaction mechanism of CT. A proposed mechanism is the release of CO2 from biotin, which subsequently abstracts a proton from the methyl group from acetyl CoA carboxylase. The resulting enolate attacks CO2 to form malonyl CoA. In a competing mechanism, proton abstraction is concerted with the attack of acetyl CoA.
Function
The function of ACC is to regulate the metabolism of fatty acids. When the enzyme is active, the product, malonyl-CoA, is produced which is a building block for new fatty acids and can inhibit the transfer of the fatty acyl group from acyl CoA to carnitine with carnitine acyltransferase, which inhibits the beta-oxidation of fatty acids in the mitochondria.
In mammals, two main isoforms of ACC are expressed, ACC1 and ACC2, which differ in both tissue distribution and function. ACC1 is found in the cytoplasm of all cells but is enriched in lipogenic tissue, such as adipose tissue and lactating mammary glands, where fatty acid synthesis is important.[10] In oxidative tissues, such as the skeletal muscle and the heart, the ratio of ACC2 expressed is higher. ACC1 and ACC2 are both highly expressed in the liver where both fatty acid oxidation and synthesis are important.[11] The differences in tissue distribution indicate that ACC1 maintains regulation of fatty acid synthesis whereas ACC2 mainly regulates fatty acid oxidation (beta oxidation).
Regulation
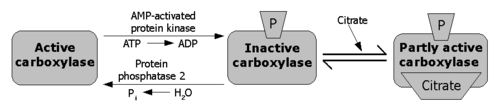
The regulation of mammalian ACC is complex, in order to control two distinct pools of malonyl CoA that direct either the inhibition of beta oxidation or the activation of lipid biosynthesis.[12]
Mammalian ACC1 and ACC2 are regulated transcriptionally by multiple promoters which mediate ACC abundance in response to the cells nutritional status. Activation of gene expression through different promoters results in alternative splicing; however, the physiological significance of specific ACC isozymes remains unclear.[11] The sensitivity to nutritional status results from the control of these promoters by transcription factors such as sterol regulatory element-binding protein 1, controlled by insulin at the transcriptional level, and ChREBP, which increases in expression with high carbohydrates diets.[13][14]
Through a feed-forward loop, citrate allosterically activates ACC.[15] Citrate may increase ACC polymerization to increase enzymatic activity; however, it is unclear if polymerization is citrate's main mechanism of increasing ACC activity or if polymerization is an artifact of in vitro experiments. Other allosteric activators include glutamate and other dicarboxylic acids.[16] Long and short chain fatty acyl CoAs are negative feedback inhibitors of ACC.[17]
Phosphorylation can result when the hormones glucagon or epinephrine bind to cell surface receptors, but the main cause of phosphorylation is due to a rise in AMP levels when the energy status of the cell is low, leading to the activation of the AMP-activated protein kinase (AMPK). AMPK is the main kinase regulator of ACC, able to phosphorylate a number of serine residues on both isoforms of ACC.[18] On ACC1, AMPK phosphorylates Ser79, Ser1200, and Ser1215. Protein kinase A also has the ability to phosphorylate ACC, with a much greater ability to phosphorylate ACC2 than ACC1. However, the physiological significance of protein kinase A in the regulation of ACC is currently unknown. Researchers hypothesize there are other ACC kinases important to its regulation as there are many other possible phosphorylation sites on ACC.[19]
When insulin binds to its receptors on the cellular membrane, it activates a phosphatase enzyme called protein phosphatase 2A (PP2A) to dephosphorylate the enzyme; thereby removing the inhibitory effect. Furthermore, insulin induces a phosphodiesterase that lowers the level of cAMP in the cell, thus inhibiting PKA, and also inhibits AMPK directly.
This protein may use the morpheein model of allosteric regulation.[20]
Clinical implications
At the juncture of lipid synthesis and oxidation pathways, ACC presents many clinical possibilities for the production of novel antibiotics and the development of new therapies for diabetes, obesity, and other manifestations of metabolic syndrome.[21] Researchers aim to take advantage of structural differences between bacterial and human ACCs to create antibiotics specific to the bacterial ACC, in efforts to minimize side effects to patients. Promising results for the usefulness of an ACC inhibitor include the finding that mice with no expression of ACC2 have continuous fatty acid oxidation, reduced body fat mass, and reduced body weight despite an increase in food consumption. These mice are also protected from diabetes.[12] A lack of ACC1 in mutant mice is lethal already at the embryonic stage. However, it is unknown whether drugs targeting ACCs in humans must be specific for ACC2.[22]
Firsocostat (formerly GS-976, ND-630, NDI-010976) is a potent allosteric ACC inhibitor, acting at the BC domain of ACC.[23] Firsocostat is under development in 2019 (Phase II)[24] by the pharmaceutical company Gilead as part of a combination treatment for non-alcoholic steatohepatitis (NASH), believed to be an increasing cause of liver failure.[25]
In addition, plant-selective ACC inhibitors are in widespread use as herbicides,[26] which suggests clinical application against Apicomplexa parasites that rely on a plant-derived ACC isoform,[27] including malaria.
See also
References
- Tong L (August 2005). "Acetyl-coenzyme A carboxylase: crucial metabolic enzyme and attractive target for drug discovery". Cellular and Molecular Life Sciences. 62 (16): 1784–803. doi:10.1007/s00018-005-5121-4. PMID 15968460.
- Brownsey RW, Zhande R, Boone AN (November 1997). "Isoforms of acetyl-CoA carboxylase: structures, regulatory properties and metabolic functions". Biochemical Society Transactions. 25 (4): 1232–8. doi:10.1042/bst0251232. PMID 9449982.
- Abu-Elheiga L, Jayakumar A, Baldini A, Chirala SS, Wakil SJ (April 1995). "Human acetyl-CoA carboxylase: characterization, molecular cloning, and evidence for two isoforms". Proceedings of the National Academy of Sciences of the United States of America. 92 (9): 4011–5. Bibcode:1995PNAS...92.4011A. doi:10.1073/pnas.92.9.4011. PMC 42092. PMID 7732023.
- Widmer J, Fassihi KS, Schlichter SC, Wheeler KS, Crute BE, King N, Nutile-McMenemy N, Noll WW, Daniel S, Ha J, Kim KH, Witters LA (June 1996). "Identification of a second human acetyl-CoA carboxylase gene". The Biochemical Journal. 316 (3): 915–22. doi:10.1042/bj3160915. PMC 1217437. PMID 8670171.
- Sasaki Y, Nagano Y (June 2004). "Plant acetyl-CoA carboxylase: structure, biosynthesis, regulation, and gene manipulation for plant breeding". Bioscience, Biotechnology, and Biochemistry. 68 (6): 1175–84. doi:10.1271/bbb.68.1175. PMID 15215578.
- "accA, acetyl-CoA carboxylase alpha subunit (Escherichia coli str. K-12 substr. MG1655)". NCBI gene. National Center for Biotechnology Information, U.S. National Library of Medicine.
- "accD, acetyl-CoA carboxylase beta subunit (Escherichia coli str. K-12 substr. MG1655)". NCBI gene. National Center for Biotechnology Information, U.S. National Library of Medicine.
- Lee CK, Cheong HK, Ryu KS, Lee JI, Lee W, Jeon YH, Cheong C (August 2008). "Biotinoyl domain of human acetyl-CoA carboxylase: Structural insights into the carboxyl transfer mechanism". Proteins. 72 (2): 613–24. doi:10.1002/prot.21952. PMID 18247344.
- Chou CY, Yu LP, Tong L (April 2009). "Crystal structure of biotin carboxylase in complex with substrates and implications for its catalytic mechanism". The Journal of Biological Chemistry. 284 (17): 11690–7. doi:10.1074/jbc.M805783200. PMC 2670172. PMID 19213731.
- Kim TS, Leahy P, Freake HC (August 1996). "Promoter usage determines tissue specific responsiveness of the rat acetyl-CoA carboxylase gene". Biochemical and Biophysical Research Communications. 225 (2): 647–53. doi:10.1006/bbrc.1996.1224. PMID 8753813.
- Barber MC, Price NT, Travers MT (March 2005). "Structure and regulation of acetyl-CoA carboxylase genes of metazoa". Biochimica et Biophysica Acta (BBA) - Molecular and Cell Biology of Lipids. 1733 (1): 1–28. doi:10.1016/j.bbalip.2004.12.001. PMID 15749055.
- Abu-Elheiga L, Matzuk MM, Abo-Hashema KA, Wakil SJ (March 2001). "Continuous fatty acid oxidation and reduced fat storage in mice lacking acetyl-CoA carboxylase 2". Science. 291 (5513): 2613–6. Bibcode:2001Sci...291.2613A. doi:10.1126/science.1056843. PMID 11283375.
- Field FJ, Born E, Murthy S, Mathur SN (December 2002). "Polyunsaturated fatty acids decrease the expression of sterol regulatory element-binding protein-1 in CaCo-2 cells: effect on fatty acid synthesis and triacylglycerol transport". The Biochemical Journal. 368 (Pt 3): 855–64. doi:10.1042/BJ20020731. PMC 1223029. PMID 12213084.
- Ishii S, Iizuka K, Miller BC, Uyeda K (November 2004). "Carbohydrate response element binding protein directly promotes lipogenic enzyme gene transcription". Proceedings of the National Academy of Sciences of the United States of America. 101 (44): 15597–602. Bibcode:2004PNAS..10115597I. doi:10.1073/pnas.0405238101. PMC 524841. PMID 15496471.
- Martin DB, Vagelos PR (June 1962). "The mechanism of tricarboxylic acid cycle regulation of fatty acid synthesis". The Journal of Biological Chemistry. 237: 1787–92. PMID 14470343.
- Boone AN, Chan A, Kulpa JE, Brownsey RW (April 2000). "Bimodal activation of acetyl-CoA carboxylase by glutamate". The Journal of Biological Chemistry. 275 (15): 10819–25. doi:10.1074/jbc.275.15.10819. PMID 10753875.
- Faergeman NJ, Knudsen J (April 1997). "Role of long-chain fatty acyl-CoA esters in the regulation of metabolism and in cell signalling". The Biochemical Journal. 323 (Pt 1): 1–12. doi:10.1042/bj3230001. PMC 1218279. PMID 9173866.
- Park SH, Gammon SR, Knippers JD, Paulsen SR, Rubink DS, Winder WW (June 2002). "Phosphorylation-activity relationships of AMPK and acetyl-CoA carboxylase in muscle". Journal of Applied Physiology. 92 (6): 2475–82. doi:10.1152/japplphysiol.00071.2002. PMID 12015362.
- Brownsey RW, Boone AN, Elliott JE, Kulpa JE, Lee WM (April 2006). "Regulation of acetyl-CoA carboxylase". Biochemical Society Transactions. 34 (Pt 2): 223–7. doi:10.1042/BST20060223. PMID 16545081.
- Selwood T, Jaffe EK (March 2012). "Dynamic dissociating homo-oligomers and the control of protein function". Archives of Biochemistry and Biophysics. 519 (2): 131–43. doi:10.1016/j.abb.2011.11.020. PMC 3298769. PMID 22182754.
- Corbett JW, Harwood JH (November 2007). "Inhibitors of mammalian acetyl-CoA carboxylase". Recent Patents on Cardiovascular Drug Discovery. 2 (3): 162–80. doi:10.2174/157489007782418928. PMID 18221116.
- Abu-Elheiga L, Matzuk MM, Kordari P, Oh W, Shaikenov T, Gu Z, Wakil SJ (August 2005). "Mutant mice lacking acetyl-CoA carboxylase 1 are embryonically lethal". Proceedings of the National Academy of Sciences of the United States of America. 102 (34): 12011–6. Bibcode:2005PNAS..10212011A. doi:10.1073/pnas.0505714102. PMC 1189351. PMID 16103361.
- Harriman G, Greenwood J, Bhat S, Huang X, Wang R, Paul D, Tong L, Saha AK, Westlin WF, Kapeller R, Harwood HJ (March 2016). "Acetyl-CoA carboxylase inhibition by ND-630 reduces hepatic steatosis, improves insulin sensitivity, and modulates dyslipidemia in rats". Proceedings of the National Academy of Sciences of the United States of America. 113 (13): E1796–805. Bibcode:2016PNAS..113E1796H. doi:10.1073/pnas.1520686113. PMC 4822632. PMID 26976583.
- Tong A (11 April 2019). "Gilead shores up hope for NASH cocktail with a glimpse at positive proof-of-concept data". Endpoints News.
- Lucas C, Lucas G, Lucas N, Krzowska-Firych J, Tomasiewicz K (September 2018). "A systematic review of the present and future of non-alcoholic fatty liver disease". Clinical and Experimental Hepatology. 4 (3): 165–174. doi:10.5114/ceh.2018.78120. PMC 6185929. PMID 30324141.
- Al-Khatib K. "Acetyl CoA Carboxylase (ACCase) Inhibitors". Herbicide Symptoms. Division of Agriculture and Natural Resources, University of California, Davis.
- Zuther E, Johnson JJ, Haselkorn R, McLeod R, Gornicki P (November 1999). "Growth of Toxoplasma gondii is inhibited by aryloxyphenoxypropionate herbicides targeting acetyl-CoA carboxylase". Proceedings of the National Academy of Sciences of the United States of America. 96 (23): 13387–92. Bibcode:1999PNAS...9613387Z. doi:10.1073/pnas.96.23.13387. PMC 23957. PMID 10557330.
Further reading
- Voet D, Voet JG (2004). Biochemistry (3rd ed.). Wiley. ISBN 978-0-471-19350-0.
- Buchanan BB, Gruissem W, Jones RL, eds. (2000). Biochemistry and molecular biology of plants. American Society of Plant Physiologists. ISBN 978-0-943088-37-2.
- Levert KL, Waldrop GL, Stephens JM (May 2002). "A biotin analog inhibits acetyl-CoA carboxylase activity and adipogenesis". The Journal of Biological Chemistry. 277 (19): 16347–50. doi:10.1074/jbc.C200113200. PMID 11907024.