Artificial heart valve
An artificial heart valve is a one-way valve implanted into the heart of a patient to replace a disfunctional native heart valve (valvular heart disease).[2][3]
Artificial heart valve | |
---|---|
![]() Different types of artificial heart valves[1] | |
Specialty | cardiology |
The human heart contains four valves: tricuspid valve, pulmonic valve, mitral valve and aortic valve. Their main purpose is to keep blood flowing in one direction through the heart, and from the heart into the major blood vessels connected to it (the pulmonary artery and the aorta). Heart valves can malfunction for a variety of reasons, which can impede the flow of blood through the valve (stenosis) and/or let blood flow backwards through the valve (regurgitation). Both processes put strain on the heart and may lead to serious problems, including heart failure.
Artificial heart valves can generally be separated into three classes: mechanical heart valve, bioprosthetic valve and tissue heart valve.
Although some dysfunctional valves can be treated with drugs or repaired, others need to be replaced with an artificial valve.[4] The main problem with artificial valves today is the regurgitation caused by form degeneration, and to repair the deformed valves, in most cases, support in the ventricle is required for any surgical operation to be performed.
Types of artificial heart valve
The three main types of artificial heart valves are mechanical, biological (bioprosthetic/tissue), and tissue-engineered valves. In the US, UK and the European Union, the most common type of artificial heart valve is the bioprosthetic valve. Mechanical valves are more commonly used in Asia and Latin America. Companies that manufacture heart valves include Edwards Lifesciences,[5] Medtronic,[6] Abbott (St. Jude Medical),[7] LivaNova,[8] CryoLife,[9] and LifeNet Health.[10]
Mechanical valves
Why Artificial heart valves
A heart contains four valves which are constantly open and closed to let blood flow into.[11] The four types of heart valves are the tricuspid, pulmonary, mitral and aortic valves. Tricuspid valve allows blood to pass through when it flows back from heart to body.[12] The pulmonary valve allows blood to flow to lungs and get oxygen.[12] The Mitral valve is where blood flows into after pickup oxygen from lungs.[12] The aortic valve is from the bottom left chamber. Blood flows into the human body from an aortic valve.[12]
There are multiple causes for heart valve damages such as age related changes, side effects from other disorders, rheumatic fever, or infections etc.[13] Other disorders include: high blood pressure and heart failure which can enlarge the heart and arteries;[13] Scar tissue caused by heart attack or injury. The common infections which can cause heart valves damage is inffective endocarditis or germs that enter the heart through the bloodstream.[13]
Types of mechanical valves
Mechanical valves come in three main types – caged ball, tilting-disc and bileaflet – with various modifications on these designs.[14] Caged ball valves are no longer implanted, but many patients are still living with this type of valve.[15] Bileaflet valves are the most common type of mechanical valve implanted in patients today.[16]
Caged ball valves
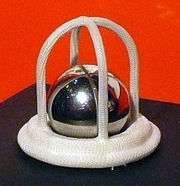
The first artificial heart valve was the caged ball valve, a type of ball check valve, in which a ball is housed inside a cage. When the heart contracts and the blood pressure in the chamber of the heart exceeds the pressure on the outside of the chamber, the ball is pushed against the cage and allows blood to flow. When the heart finishes contracting, the pressure inside the chamber drops and the ball moves back against the base of the valve forming a seal.
In 1952, Charles A. Hufnagel implanted caged ball heart valves into ten patients (six of whom survived the operation), marking the first long-term success in prosthetic heart valves.[17] A similar valve was invented by Miles 'Lowell' Edwards and Albert Starr in 1960 (commonly referred to as the Starr-Edwards silastic ball valve).[18] This consisted of a silicone ball enclosed in a methyl metacrylate cage welded to a ring. The Starr-Edwards valve was first implanted in a human on August 25, 1960,[18] but was discontinued by Edwards Lifesciences in 2007.
Caged ball valves are strongly associated with blood clot formation, so patients who have one required a high degree of anticoagulation, usually with a target INR of 3.0–4.5.[19]
Tilting-disc valves
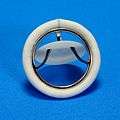
Introduced in 1969, the first clinically available tilting-disc valve was the Bjork-Shiley valve.[20] Tilting‑disc valves, a type of swing check valve, are made of a metal ring covered by an ePTFE fabric. The metal ring holds, by means of two metal supports, a disc that opens when the heart beats to let blood flow through, then closes again to prevent blood flowing backwards. The disc is usually made of an extremely hard carbon material (pyrolytic carbon), enabling the valve to function for years without wearing out.
Bileaflet valves
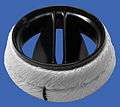
Introduced in 1979, bileaflet valves are made of two semicircular leaflets that revolve around struts attached to the valve housing.[21] With a larger opening than caged ball or tilting-disc valves, they carry a lower risk of blood clots.[21] They are, however, vulnerable to blood backflow.[22]
Advantages of mechanical valves
The major advantage of mechanical valves over bioprosthetic valves is their greater durability.[23] Made from metal and/or pyrolytic carbon,[14] they can last 20–30 years.[23]
Disadvantages of mechanical valves
Blood damage
One of the major drawbacks of mechanical heart valves is that they are associated with an increased risk of blood clots. Clots formed by red blood cell and platelet damage can block blood vessels leading to serious consequences. People with mechanical valves need to take anticoagulants (blood thinners), such as warfarin, for the rest of their life.[23]
Mechanical heart valves can also cause mechanical hemolytic anemia, a condition where the red blood cells are damaged as they pass through the valve.[24]
Noise
Some patients with mechanical valves can hear clicks as their valve closes, which some find disturbing.[25]
Cavitation
Cavitation is the rapid formation of vaporous microbubbles in a fluid due to a local drop of pressure below the vaporization pressure at a given temperature. Cavitation in the blood can lead to mechanical heart valve failure,[26] so cavitation testing is an essential part of the valve design verification process.
Fluid mechanics
Many of the complications associated with mechanical heart valves can be explained through fluid mechanics. For example, blood clot formation is a side effect of high shear stresses created by the design of the valves. From an engineering perspective, an ideal heart valve would produce minimal pressure drops, have small regurgitation volumes, minimize turbulence, reduce prevalence of high stresses, and not create flow separations in the vicinity of the valve.
Foreign body rejection and lack of adaptation to growth
Implanting mechanical valves will cause foregin body rejection. The blood may coagulate and eventually result in a hemostasis. The usage of anticoagulation drugs will be interminable to prevent thrombosis.[27] The side effect of Heparin, the most commonly used anticoagulant, includes hardship of forming blood clots and can cause major bleeding. It may also cause Heparin induced thrombocytopenia (HIT), heparin-associated osteoporosis (HAO) and skin allergies.[28]
Mechanical valves sometimes cause infections. When infections happen it would be very hard to cure unless changing the heart valves.
Mechanical valves are not able to adapt to the changes like physical growth of the body. The patient will have to get the valves replaced if the size of their heart changes [4].
Bioprosthetic (tissue) valves
Bioprosthetic valves are usually made from animal tissue (heterograft/xenograft) mounted on a metal or polymer support.[15] Bovine (cow) tissue is most commonly used, but some are made from porcine (pig) tissue.[29] The tissue is treated to prevent rejection and calcification (where calcium builds up on the replacement valve and stops it working properly).[30]
Occasionally, alternatives to animal tissue valves are used: aortic homografts and pulmonary autografts. An aortic homograft is an aortic valve from a human donor, retrieved either after their death or from their heart if they are undergoing a heart transplant.[16] A pulmonary autograft, also known as the Ross procedure is where the aortic valve is removed and replaced with the patient's own pulmonary valve (the valve between the right ventricle and the pulmonary artery). A pulmonary homograft (a pulmonary valve taken from a cadaver) is then used to replace the patient's own pulmonary valve. This procedure was first performed in 1967 and is used primarily in children, as it allows the patient's own pulmonary valve (now in the aortic position) to grow with the child.[16]
Advantages of bioprosthetic heart valves
Bioprosthetic valves are less likely than mechanical valves to cause blood clots, so do not require lifelong anticoagulation. As a result, people with bioprosthetic valves have a lower risk of bleeding that those with mechanical valves.[23]
Disadvantages of bioprosthetic heart valves
Tissue valves are less durable than mechanical valves, typically lasting 10–20 years.[31] This means that people with bioprosthetic valves have a higher risk of requiring another aortic valve replacement in their lifetime.[23] Bioprosthetic valves tend to deteriorate more quickly in younger patients.[32]
Recently, scientists have developed a new advanced tissue preservation technology, with the aim of improving the durability of bioprosthetic valves. In sheep and rabbit studies, tissue preserved using this new technology (called RESILIA™ tissue) had less calcification than control tissue.[33][34] A valve containing this tissue is now marketed, but long-term durability data in patients are not yet available.[35]
Current bioprosthetic valves lack longevity, and will calcify as time flows.[36] The valves will make the valve cusp become stiff and thick that cannot close completely when the valves calcify.[36] Moreover, bioprosthetic valves can’t grow with or adapt to the patient: if a child has bioprosthetic valves they will need to get the valves replaced several times to fit their physical growth.[36]
Tissue-engineered valves
For over 30 years researchers have been trying to grow heart valves in vitro.[37] These tissue‑engineered valves involve seeding human cells on to a scaffold.[37] The two main types of scaffold are natural scaffolds, such as decellularized tissue, or scaffolds made from degradable polymers.[38] The scaffold acts as an extracellular matrix, guiding tissue growth into the correct 3D structure of the heart valve.[38][37] Some tissue-engineered heart valves have been tested in clinical trials,[38] but none are commercially available.
Modeling and 3D printing of heart valves
Usually the tissue engineered heart valves are patient specific and will need to have a pre-design.[39] Modelling will enable the doctor to design appropriate heart valves based on the patient’s physical status. 3D printing is used because of its high accuracy and precision of dealing with different biomaterials.[39] 3D printing can help with minimizing the error that may occur when producing the valves. The structure of the valves is very important to make it functioning properly, and 3D printing is the best way to produce these structures.[39] The most important technique of 3D printing in making a heart valve is microstamping. It allows the cells to be arranged in the predesigned 3D structure, which enables the valves to function.[39]
Nanofiber as the scaffold of tissue engineered valves
Nanofiber is a biodegradable and synthesized material. It is able to provide a good framework for the cells to grow.[40] The surface of nanofiber provides a suitable environment for the cells to adhere. Even though most of the drugs have a long protein chain, they can still stick well on the surface of nanofiber[40] Therefore, nanofibers are used as scaffolds for musculoskeletal tissue engineering. and as a carrier for the controlled release of drugs, DNA, and proteins.[28]
Types of cells used in tissue engineering
Cells that are used for tissue engineered heart valves are expected to secrete the extracellular matrix (ECM).[12] Extracellular matrix provides support to maintain the shape of the valves and determines the cell activities.[41] There are three types of cells commonly used to produce heart valves: stem cells, progenitor cells and differentiated cells.[12] These cells can be found in the individual patients’ bodies or from their bloodstream to maximize the adaptability of the valves.[12]
Challenges that tissue engineered valves are facing
Scientists now can follow the structure of heart valves to produce something that looks similar to them.[42] However, since the tissue engineered valves lack of living cells, they either fail to perform their functions like natural heart valves, or they are functioning when they are implanted, but gradually degrade over time.[42] It is hardly possible to fix cell degrading without replacing the heart valves. Scientists are researching on producing durable tissue engineered valves but until now only natural valves can be both functioning and adapting.[42]
Future of tissue engineering valves
Tissue engineered heart valves are aiming to achieve these goals: anti‐thrombogenic, biocompatible, durable, resistant to calcification, and exhibits a physiological hemodynamic profile.[43] The valve should also grow with the human body.[43]
To achieve these goals, the scaffold should be carefully chosen—there are three main candidates: decellularized ECM (xenografts or homografts), natural polymers, and synthetic polymers.[43] To select the most suitable material, we should consider the following aspects: 1. The interaction of cells and scaffold. 2. Fabrication of the materials and the impact on the 3D structure. 3. The durability of the scaffold.[43]
Difference between mechanical and tissue valves
Mechanical and tissue valves are made by different materials. The materials used in making mechanical valves are titanium and carbon.[44] Tissue valves are made up of human or animal tissue. The valves composed of human tissue, known as allografts or homografts, are from donors' human hearts.[44] The valves made from animal tissues, such as the porcine (pig), bovine (cow) and equine (horse) models, are secured with a preserving solution.[44]
The structure of both valves are different too. The mechanical valves generally include two leaflets and a ring of knitted fabric encircling a metal ring.[44] The tissue valves instead are enclosed by a ring of knitted fabric which is sewn onto the heart.[44]
Choosing Artificial Hearts
Choosing artificial valves are depending on factors such as patients’ age, physical condition, other medications etc.[45] Patients should always follow doctors' recommendations in selecting artificial valves.
Mechanical valves can be a better choice for younger people(younger than 65) and people at risk of valve deterioration due to its durability.[45] It is also preferable to patients who are already taking warfarin and people who would be at risk for another valve replacement operation.[45]
Tissue valves are better for older age groups as another valve replacement operation may not be needed in their lifetime. Due to the risk of forming blood clots for mechanical valves and severe bleeding as a major side effect of taking blood-thinning medications, people who have a risk of blood bleeding and are not willing to take warfarin may also consider tissue valves.[45] Other patients who may be more suitable for tissue valves are the ones who have other planned surgeries and are not willing to take blood-thinning medications. People who have pregnancy plans may also consider tissue valves as there is a risk for warfarin for pregnancy.[45]
Functional requirements of artificial heart valves
An artificial heart valve should ideally function like a natural heart valve.[15] The functioning of natural heart valves is characterized by many advantages:
- Minimal regurgitation – This means that the amount of blood leaking backwards through the valve as it closes is small. Some degree of valvular regurgitation is inevitable and natural, up to around 5 ml per beat.[46] However, several heart valve pathologies (e.g. rheumatic endocarditis) may lead to clinically significant valvular regurgitation. A desirable characteristic of heart valve prostheses is that regurgitation is minimal over the full range of physiological heart function.
- Minimal transvalvular pressure gradient – Whenever a fluid flows through a restriction, such as a valve, a pressure gradient arises over the restriction. This pressure gradient is a result of the increased resistance to flow through the restriction. Natural heart valves have a low transvalvular pressure gradient as they present little obstruction to the flow through themselves, normally less than 16 mmHg. A desirable characteristic of heart valve prostheses is that their transvalvular pressure gradient is as small as possible.
- Non-thrombogenic – Natural heart valves are lined with an endothelium comparable with the endothelium lining the heart chambers, so they are not normally thrombogenic (i.e. they don't cause blood clots). Blood clots can be hazardous because they can lodge in, and block, downstream arteries (e.g. coronary arteries, leading to heart attack [myocardial infarction]; or cerebral arteries, leading to stroke). A desirable characteristic of artificial heart valves is that they are non- or minimally thrombogenic.
- Self-repairing – Valve leaflets retain some capacity for repair thanks to regenerative cells (e.g. fibroblasts) in the connective tissue from which the leaflets are composed. As the human heart beats approximately 3.4×109 times during a typical human lifespan, this limited but nevertheless present repair capacity is critically important. No heart valve prostheses can currently self-repair, but tissue-engineered valves may eventually offer such capabilities.[38]
- Rapid dynamic response
Artificial Heart Valve Repair
Artificial heart valves are expected to last from 10 to 30 years, and by the time, the patient will often have a new heart valve installed.[47]
The most common problems with artificial heart valves are various forms of degeneration, including gross billowing of leaflets, ischemic mitral valve pathology, and minor chordal lengthening.[12]
The repairing process of the artificial heart valve regurgitation (mitral or tricuspid regurgitation) and stenosis usually requires the surgeon for an open-heart surgery, and a repair or partial replacement of regurgitant valves, such as mitral valve, is usually preferred.[12] There have been numerous inventions developed in this field for proper artificial heart valve repair.
A general method of supporting an artificial heart valve according to the current invention, such as a distance adjusting device within the valve, the supporting design is first linked with and then anchored at the annulus of the valve, or it may already have an extending pillar for anchor.[48] For most cases, in the deployed state, the anchor is positioned in perpendicular to the longitudinal axis.[49] To open and close the leaflet, the pillar is then connected to one of the valve leaflets for supporting. With all kinds of repairing apparatus, the pillar may be positioned along with a flexible tensile member, for example, an natural or artificial rope. Or a type of direct connection involves extending a coil from the pillar into the two nearby leaflets to bridge with the center parts of the leaflets. Other forms of connections for supporting purpose also involve artificial chord or pillar attachments described above.[48]
Although the methods of the current inventions are aimed at contracting the artificial heart valve’s annulus, particularly for the treatment of valve regurgitation, these inventions may find wider applications. Especially, the clip deployment device can be used in other circumstances, such as to transfer a plurality of sequential clips in a straight or curved manner to tighten the tissue or optionally bind the tether portion of the tissue.[12]
From the current inventions, their methods and systems are generally designed for convenience in transvascular, minimally invasive and other surgical procedures by improving the efficiency of delivery of the therapeutic apparatus.[49]
Researchers are developing catheter based surgery that repairs the artificial heart valve without cutting for any incision, also referred to as “percutaneous”, and also utilizing polymers to produce flexible leaflet valves, while inheriting the advantages of current commercial bioprosthetic and mechanical valves.[50]
Additional images
- 3D Rendering of Mechanical Valve
- 3D Rendering of Mechanical Valve (St. Francis model)
See also
- Artificial heart
- Artificial Total Heart
- The total artificial heart is a dual-ventricular, pneumatic, pulsatile blood pump that will be transplanted to substitute for the ventricle and its four heart valves.[51]
- The total artificial heart (TAH) device, for now, can only be approved by the FDA for biventricular heart failure, and this is only to earn time for the patient for a heart transplantation.[52]
- In case of an irreversible biventricular failure, TAH can greatly increase the chance of survival compared to cardiac valves, and the survival rates for one-year and five-year after the successful transplantation are 86% and 64%.[51]
- The patient's hemodynamic status was alleviated with implantation of a total heart, and the cardiac index increased from the baseline value of 1.9 to 3.2 liters per square meter.[51]
- Not only promoting immediate hemodynamic recovery and clinical stability, the implantation of the total artificial heart improves the prognosis of the dying patient, leading to the restority of the end-organs, which ultimately prepares the patient for cardiac transplantation.[51]
- The total artificial heart has served as an emergent rescue device, and it fails in long term usage because it might cause hemolysis and thrombosis. Due to the inflexible structure of TAH that would lead to blood clot, bleeding, infection, and organ failures are the most common obstacles.[52]
- Experiment on continuous-flow TAH shows a patient’s pulseless circulation without affecting homeostasis or exercise.[53]
- The continuous-flow TAH is more durable, responsive, compatible for the human body compared to a conventional TAH.[53]
- Artificial Total Heart
- Mitral valve replacement
- Aortic valve replacement
References
- Kostrzewa, B; Rybak, Z (2013). "History, present and future of biomaterials used for artificial heart valves". Polimery W Medycynie. 43 (3): 183–189. PMID 24377185.
- Bertazzo, Sergio; Gentleman, Eileen; Cloyd, Kristy L.; Chester, Adrian H.; Yacoub, Magdi H.; Stevens, Molly M. (2013). "Nano-analytical electron microscopy reveals fundamental insights into human cardiovascular tissue calcification". Nature Materials. 12 (6): 576–583. Bibcode:2013NatMa..12..576B. doi:10.1038/nmat3627. PMC 5833942. PMID 23603848.
- Miller, Jordan D. (2013). "Cardiovascular calcification: Orbicular origins". Nature Materials. 12 (6): 476–478. Bibcode:2013NatMa..12..476M. doi:10.1038/nmat3663. PMID 23695741.
- Baumgartner, Helmut; Falk, Volkmar; Bax, Jeroen J; De Bonis, Michele; Hamm, Christian; Holm, Per Johan; Iung, Bernard; Lancellotti, Patrizio; Lansac, Emmanuel (2017-09-21). "2017 ESC/EACTS Guidelines for the management of valvular heart disease". European Heart Journal. 38 (36): 2739–2791. doi:10.1093/eurheartj/ehx391. ISSN 0195-668X. PMID 28886619.
- "Surgical aortic heart valves | Edwards Lifesciences". www.edwards.com. Retrieved 2019-07-29.
- Medtronic. "Heart Valve Therapies - Surgical Replacement". www.medtronic.com. Retrieved 2019-07-29.
- "Surgical Valves | Trifecta GT Valve and Epic Mitral Valve". www.cardiovascular.abbott. Retrieved 2019-07-29.
- "LivaNova". www.livanova.com. Retrieved 2019-07-29.
- "On-X Heart Valves". CryoLife, Inc. Retrieved 2019-07-29.
- "Cardiac | LifeNet Health". www.lifenethealth.org. Retrieved 2019-07-29.
- "Heart Valve Replacement: Which Type Is Best for You?". Health Essentials from Cleveland Clinic. 2018-06-14. Retrieved 2020-08-04.
- Hasan, Anwarul; Saliba, John; Pezeshgi Modarres, Hassan; Bakhaty, Ahmed; Nasajpour, Amir; Mofrad, Mohammad R. K.; Sanati-Nezhad, Amir (2016-10-01). "Micro and nanotechnologies in heart valve tissue engineering". Biomaterials. 103: 278–292. doi:10.1016/j.biomaterials.2016.07.001. ISSN 0142-9612.
- Muraru, Denisa; Anwar, Ashraf M.; Song, Jae-Kwan (December 2016). "Heart valve disease: tricuspid valve disease". Oxford Medicine Online. doi:10.1093/med/9780198726012.003.0037.
- Gott, Vincent L; Alejo, Diane E; Cameron, Duke E (2003). "Mechanical heart valves: 50 years of evolution". The Annals of Thoracic Surgery. 76 (6): S2230–S2239. doi:10.1016/j.athoracsur.2003.09.002. ISSN 0003-4975. PMID 14667692.
- Pibarot, Philippe; Dumesnil, Jean G. (2009-02-24). "Prosthetic Heart Valves: Selection of the Optimal Prosthesis and Long-Term Management". Circulation. 119 (7): 1034–1048. doi:10.1161/CIRCULATIONAHA.108.778886. ISSN 0009-7322. PMID 19237674.
- Bloomfield, P. (2002-06-01). "Choice of heart valve prosthesis". Heart. 87 (6): 583–589. doi:10.1136/heart.87.6.583. PMC 1767148. PMID 12010950.
- Rajashekar. "Development of mechanical heart valves - an inspiring tale". www.j-pcs.org. Retrieved 2019-02-05.
- Matthews, A M (1998). "The development of the Starr-Edwards heart valve". Texas Heart Institute Journal. 25 (4): 282–293. ISSN 0730-2347. PMC 325574. PMID 9885105.
- Goldsmith, Ira; Turpie, Alexander G G; Lip, Gregory Y H (2002-11-23). "Valvar heart disease and prosthetic heart valves". BMJ : British Medical Journal. 325 (7374): 1228–1231. doi:10.1136/bmj.325.7374.1228. ISSN 0959-8138. PMC 1124694. PMID 12446543.
- Sun, Jack CJ; Davidson, Michael J; Lamy, Andre; Eikelboom, John W (2009). "Antithrombotic management of patients with prosthetic heart valves: current evidence and future trends". The Lancet. 374 (9689): 565–576. doi:10.1016/S0140-6736(09)60780-7. PMID 19683642.
- Rajashekar, P (2015). "Development of mechanical heart valves - an inspiring tale". Journal of the Practice of Cardiovascular Sciences. 1 (3): 289. doi:10.4103/2395-5414.177309. ISSN 2395-5414.
- Ohta, Yuji; Kikuta, Yukiaki; Shimooka, Toshiyuki; Mitamura, Yoshinori; Yuhta, Toshio; Dohi, Takeyoshi (2000). "Effect of the Sinus of Valsalva on the Closing Motion of Bileaflet Prosthetic Heart Valves". Artificial Organs. 24 (4): 309–312. doi:10.1046/j.1525-1594.2000.06534-2.x. ISSN 0160-564X. PMID 10816206.
- Tillquist; Tillquist; Maddox, Tom (2011). "Cardiac crossroads: deciding between mechanical or bioprosthetic heart valve replacement". Patient Preference and Adherence. 5: 91–9. doi:10.2147/PPA.S16420. ISSN 1177-889X. PMC 3063655. PMID 21448466.
- Pooja, Sethi; Murtaza, Ghulam; Rahman, Zia; Zaidi, Syed; Helton, Thomas; Paul, Timir (2017-04-08). "Valvular Hemolysis Masquerading as Prosthetic Valve Stenosis". Cureus. 9 (4): e1143. doi:10.7759/cureus.1143. ISSN 2168-8184. PMC 5422110. PMID 28491484.
- Koertke, H (2003). "Does the noise of mechanical heart valve prostheses affect quality of life as measured by the SF-36® questionnaire?". European Journal of Cardio-Thoracic Surgery. 24 (1): 52–58. doi:10.1016/s1010-7940(03)00172-6. ISSN 1010-7940. PMID 12853045.
- Johansen, Peter (2004). "Mechanical heart valve cavitation". Expert Review of Medical Devices. 1 (1): 95–104. doi:10.1586/17434440.1.1.95. ISSN 1743-4440. PMID 16293013.
- Namdari, Mehrdad; Eatemadi, Ali (2016-12-01). "Nanofibrous bioengineered heart valve—Application in paediatric medicine". Biomedicine & Pharmacotherapy. 84: 1179–1188. doi:10.1016/j.biopha.2016.10.058. ISSN 0753-3322.
- Walenga, Jeanine M.; Bick, Rodger L. (1998-05-01). "HEPARIN-INDUCED THROMBOCYTOPENIA, PARADOXICAL THROMBOEMBOLISM, AND OTHER SIDE EFFECTS OF HEPARIN THERAPY". Medical Clinics of North America. 82 (3): 635–658. doi:10.1016/S0025-7125(05)70015-8. ISSN 0025-7125.
- Hickey, Graeme L.; Grant, Stuart W.; Bridgewater, Ben; Kendall, Simon; Bryan, Alan J.; Kuo, James; Dunning, Joel (2015). "A comparison of outcomes between bovine pericardial and porcine valves in 38 040 patients in England and Wales over 10 years". European Journal of Cardio-Thoracic Surgery. 47 (6): 1067–1074. doi:10.1093/ejcts/ezu307. ISSN 1873-734X. PMID 25189704.
- Li, Kan Yan Chloe (2019-04-11). "Bioprosthetic Heart Valves: Upgrading a 50-Year Old Technology". Frontiers in Cardiovascular Medicine. 6: 47. doi:10.3389/fcvm.2019.00047. ISSN 2297-055X. PMC 6470412. PMID 31032263.
- Harris, Christopher; Croce, Beth; Cao, Christopher (2015-10-07). "Tissue and mechanical heart valves". Annals of Cardiothoracic Surgery. 4 (4): 399. doi:10.3978/6884 (inactive 2020-06-03). ISSN 2225-319X. PMC 4526499. PMID 26309855.
- Johnston, Douglas R.; Soltesz, Edward G.; Vakil, Nakul; Rajeswaran, Jeevanantham; Roselli, Eric E.; Sabik, Joseph F.; Smedira, Nicholas G.; Svensson, Lars G.; Lytle, Bruce W. (2015). "Long-Term Durability of Bioprosthetic Aortic Valves: Implications From 12,569 Implants". The Annals of Thoracic Surgery. 99 (4): 1239–1247. doi:10.1016/j.athoracsur.2014.10.070. PMC 5132179. PMID 25662439.
- Flameng, Willem; Hermans, Hadewich; Verbeken, Erik; Meuris, Bart (2015). "A randomized assessment of an advanced tissue preservation technology in the juvenile sheep model". The Journal of Thoracic and Cardiovascular Surgery. 149 (1): 340–345. doi:10.1016/j.jtcvs.2014.09.062. ISSN 0022-5223. PMID 25439467.
- Shang, Hao; Claessens, Steven M.; Tian, Bin; Wright, Gregory A. (2016-12-20). "Aldehyde reduction in a novel pericardial tissue reduces calcification using rabbit intramuscular model". Journal of Materials Science: Materials in Medicine. 28 (1): 16. doi:10.1007/s10856-016-5829-8. ISSN 0957-4530. PMC 5174141. PMID 28000112.
- Bartuś, Krzysztof; Litwinowicz, Radosław; Kuśmierczyk, Mariusz; Bilewska, Agata; Bochenek, Maciej; Stąpór, Maciej; Woźniak, Sebastian; Różański, Jacek; Sadowski, Jerzy (2017-12-19). "Primary safety and effectiveness feasibility study after surgical aortic valve replacement with a new generation bioprosthesis: one-year outcomes". Kardiologia Polska. 76 (3): 618–624. doi:10.5603/KP.a2017.0262. ISSN 0022-9032. PMID 29297188. S2CID 4061454.
- Hasan, Anwarul; Saliba, John; Pezeshgi Modarres, Hassan; Bakhaty, Ahmed; Nasajpour, Amir; Mofrad, Mohammad R. K.; Sanati-Nezhad, Amir (2016-10-01). "Micro and nanotechnologies in heart valve tissue engineering". Biomaterials. 103: 278–292. doi:10.1016/j.biomaterials.2016.07.001. ISSN 0142-9612.
- Stassen, O. M. J. A.; Muylaert, D. E. P.; Bouten, C. V. C.; Hjortnaes, J. (2017). "Current Challenges in Translating Tissue-Engineered Heart Valves". Current Treatment Options in Cardiovascular Medicine. 19 (9): 71. doi:10.1007/s11936-017-0566-y. ISSN 1092-8464. PMC 5545463. PMID 28782083.
- Blum, Kevin M.; Drews, Joseph D.; Breuer, Christopher K. (2018). "Tissue-Engineered Heart Valves: A Call for Mechanistic Studies". Tissue Engineering Part B: Reviews. 24 (3): 240–253. doi:10.1089/ten.teb.2017.0425. ISSN 1937-3368. PMC 5994154. PMID 29327671.
- Theus, Andrea S.; Tomov, Martin L.; Cetnar, Alex; Lima, Bryanna; Nish, Joy; McCoy, Kevin; Mahmoudi, Morteza; Serpooshan, Vahid (2019-06-01). "Biomaterial approaches for cardiovascular tissue engineering". Emergent Materials. 2 (2): 193–207. doi:10.1007/s42247-019-00039-3. ISSN 2522-574X.
- Namdari, Mehrdad; Eatemadi, Ali (2016-12-01). "Nanofibrous bioengineered heart valve—Application in paediatric medicine". Biomedicine & Pharmacotherapy. 84: 1179–1188. doi:10.1016/j.biopha.2016.10.058. ISSN 0753-3322.
- Hay, E. D. (2013-11-11). Cell Biology of Extracellular Matrix: Second Edition. Springer Science & Business Media. ISBN 978-1-4615-3770-0.
- Chester, Adrian H.; Grande-Allen, K. Jane (2020-04-21). "Which Biological Properties of Heart Valves Are Relevant to Tissue Engineering?". Frontiers in Cardiovascular Medicine. 7. doi:10.3389/fcvm.2020.00063. ISSN 2297-055X. PMC 7186395. PMID 32373630.
- Nachlas, Aline L. Y.; Li, Siyi; Davis, Michael E. (2017). "Developing a Clinically Relevant Tissue Engineered Heart Valve—A Review of Current Approaches". Advanced Healthcare Materials. 6 (24): 1700918. doi:10.1002/adhm.201700918. ISSN 2192-2659.
- Schmidt, Jillian B.; Tranquillo, Robert T. (2013), "Tissue-Engineered Heart Valves", Heart Valves, Boston, MA: Springer US, pp. 261–280, ISBN 978-1-4614-6143-2, retrieved 2020-08-04
- Chambers, John B.; Vanoverschelde, Jean-Louis (March 2011). "Replacement heart valves". Oxford Medicine Online. doi:10.1093/med/9780199599639.003.0017.
- Kasegawa, H; Iwasaki, K; Kusunose, S; Tatusta, R; Doi, T; Yasuda, H; Umezu, M (2012). "Assessment of a novel stentless mitral valve using a pulsatile mitral valve simulator". The Journal of Heart Valve Disease. 21 (1): 71–75. PMID 22474745.
- , "Devices and methods for heart valve repair", issued 2003-06-13
- , "Heart valve repair apparatus and methods", issued 2002-10-09
- , "Delivery devices and methods for heart valve repair", issued 2004-07-27
- Bezuidenhout, Deon; Williams, David F.; Zilla, Peter (2015-01-01). "Polymeric heart valves for surgical implantation, catheter-based technologies and heart assist devices". Biomaterials. 36: 6–25. doi:10.1016/j.biomaterials.2014.09.013. ISSN 0142-9612.
- Copeland, Jack G.; Smith, Richard G.; Arabia, Francisco A.; Nolan, Paul E.; Sethi, Gulshan K.; Tsau, Pei H.; McClellan, Douglas; Slepian, Marvin J. (2004-08-26). "Cardiac Replacement with a Total Artificial Heart as a Bridge to Transplantation". New England Journal of Medicine. 351 (9): 859–867. doi:10.1056/NEJMoa040186. ISSN 0028-4793. PMID 15329423.
- "SynCardia 70cc TAH-t for Destination Therapy (DT) - Full Text View - ClinicalTrials.gov". clinicaltrials.gov. Retrieved 2020-08-04.
- Frazier, O. H.; Cohn, William E.; Tuzun, Egemen; Winkler, Jo Anna; Gregoric, Igor D. (2009). "Continuous-Flow Total Artificial Heart Supports Long-Term Survival of a Calf". Texas Heart Institute Journal. 36 (6): 568–574. ISSN 0730-2347. PMC 2801939. PMID 20069083.
Further reading
- Bendet, IaA; Morozov, SM; Skumin, VA (1980). "Psychological aspects of the rehabilitation of patients after the surgical treatment of heart defects" Психологические аспекты реабилитации больных после хирургического лечения пороков сердца [Psychological aspects of the rehabilitation of patients after the surgical treatment of heart defects]. Kardiologiia (in Russian). 20 (6): 45–51. PMID 7392405.
- Skumin, VA (1979). "Nurse's role in medico-psychological rehabilitation of patients with artificial heart valves". Meditsinskaia Sestra. 38 (9): 44–45. PMID 259874.
- Skumin, VA (1982). "Nonpsychotic mental disorders in patients with acquired heart defects before and after surgery (review)". Zhurnal Nevropatologii I Psikhiatrii Imeni S.S. Korsakova. 82 (11): 130–5. PMID 6758444.
- Klepetko, W; Moritz, A; Mlczoch, J; Schurawitzki, H; Domanig, E; Wolner, E (1989). "Leaflet fracture in Edwards-Duromedics bileaflet valves". The Journal of Thoracic and Cardiovascular Surgery. 97 (1): 90–94. doi:10.1016/S0022-5223(19)35130-X. PMID 2911200.
- Podesser, BK; Khuenl-Brady, G; Eigenbauer, E; Roedler, S; Schmiedberger, A; Wolner, E; Moritz, A (1998). "Long-term results of heart valve replacement with the Edwards Duromedics bileaflet prosthesis: A prospective ten-year clinical follow-up". The Journal of Thoracic and Cardiovascular Surgery. 115 (5): 1121–1129. doi:10.1016/s0022-5223(98)70412-x. PMID 9605082.
- Knapp RJ, Daily JW, Hammitt FG (1970). Cavitation. New York: McGraw-Hill Int. Book Co.
- Lim, WL; Chew, YT; Low, HT; Foo, WL (2003). "Cavitation phenomena in mechanical heart valves: The role of squeeze flow velocity and contact area on cavitation initiation between two impinging rods". Journal of Biomechanics. 36 (9): 1269–1280. doi:10.1016/s0021-9290(03)00161-1. PMID 12893035.
- Bluestein, D; Einav, S; Hwang, NH (1994). "A squeeze flow phenomenon at the closing of a bileaflet mechanical heart valve prosthesis". Journal of Biomechanics. 27 (11): 1369–78. doi:10.1016/0021-9290(94)90046-9. PMID 7798287.
- Graf, T; Fischer, H; Reul, H; Rau, G (1991). "Cavitation potential of mechanical heart valve prostheses". The International Journal of Artificial Organs. 14 (3): 169–174. doi:10.1177/039139889101400309. PMID 2045192.
- Kafesjian, R; Wieting, DW; Ely, J; Chahine, GL; Frederick, GS; Watson, RE (1990). "Characterization of Cavitation Potential of Pyrolitic Carbon". In Bodnar, Endré (ed.). Surgery for Heart Valve Disease: Proceedings of the 1989 Symposium. ICR. pp. 509–16. ISBN 978-1-872743-00-4.
- Chahine, GL (1996). "Scaling of mechanical heart valves for cavitation inception: Observation and acoustic detection". The Journal of Heart Valve Disease. 5 (2): 207–215. PMID 8665016.
- Zapanta, CM; Stinebring, DR; Sneckenberger, DS; Deutsch, S; Geselowitz, DB; Tarbell, JM; Synder, AJ; Rosenberg, G; Weiss, WJ; Pae, WE; Pierce, WS (1996). "In vivo observation of cavitation on prosthetic heart valves". ASAIO Journal. 42 (5): M550–5. doi:10.1097/00002480-199609000-00047. PMID 8944940.
- Richard, G; Beavan, A; Strzepa, P (1994). "Cavitation threshold ranking and erosion characteristics of bileaflet heart valve prostheses". The Journal of Heart Valve Disease. 3 Suppl 1: S94–101. PMID 8061875.
External links
- "Page describing types of heart valve replacements". Archived from the original on 13 November 2006.
- "New Design for Mechanical Heart Valves". ScienceDaily. 23 November 2011.