Laser cooling
Laser cooling includes a number of techniques in which atomic and molecular samples are cooled down to near absolute zero. Laser cooling techniques rely on the fact that when an object (usually an atom) absorbs and re-emits a photon (a particle of light) its momentum changes. For an ensemble of particles, their thermodynamic temperature is proportional to the variance in their velocity. That is, more homogeneous velocities among particles corresponds to a lower temperature. Laser cooling techniques combine atomic spectroscopy with the aforementioned mechanical effect of light to compress the velocity distribution of an ensemble of particles, thereby cooling the particles.

1 | A stationary atom sees the laser neither red- nor blue-shifted and does not absorb the photon. |
---|---|
2 | An atom moving away from the laser sees it red-shifted and does not absorb the photon. |
3.1 | An atom moving towards the laser sees it blue-shifted and absorbs the photon, slowing the atom. |
3.2 | The photon excites the atom, moving an electron to a higher quantum state. |
3.3 | The atom re-emits a photon. As its direction is random, there is no net change in momentum over many absorption-emission cycles. |
The first example of laser cooling, and also still the most common method (so much so that it is still often referred to simply as 'laser cooling') is Doppler cooling. Other methods of laser cooling include:
- Sisyphus cooling[1]
- Resolved sideband cooling
- Raman sideband cooling
- Velocity selective coherent population trapping (VSCPT)[2]
- Gray molasses
- Cavity mediated cooling [3]
- Use of a Zeeman slower
- Electromagnetically induced transparency (EIT) cooling[4]
History
Early attempts
At the advent of laser cooling techniques, Maxwell's theory of electromagnetism had already led to the quantification of electromagnetic radiation exerting a force (radiation pressure), however it wasn't until the turn of the twentieth century when studies by Lebedev (1901), Nichols (1901), and Hull (1903) experimentally demonstrated that force.[5] Following that period, in 1933, Frisch exemplified the pressure exerted on atoms by light. Starting in the early 1970s, lasers were then utilized to further explore atom manipulation. The introduction of lasers in atomic manipulation experiments acted as the advent of laser cooling proposals in the mid 1970s. Laser cooling was separately introduced in 1975 by two different research groups: Hänsch and Schawlow, and Wineland and Dehmelt. They both outlined a process of slowing heat-based velocity in atoms by "radiative forces."[6] In the paper by Hänsch and Schawlow, the effect of radiation pressure on any object that reflects light is described. That concept was then connected to the cooling of atoms in a gas.[7] These early proposals for laser cooling only relied on "scattering force", the name for the radiation pressure. In later proposals, laser trapping, a variant of cooling which requires both scattering and a dipole force, would be introduced.[6]
In the late 70s, Ashkin described how radiation forces can be used to both optically trap atoms and simultaneously cool them.[5] He emphasized how this process could allow for long spectroscopic measurements without the atoms escaping the trap and proposed the overlapping of optical traps in order to study interactions between different atoms.[8] Closely following Ashkin's letter in 1978, two research groups: Wineland, Drullinger and Walls, and Neuhauser, Hohenstatt, Toscheck and Dehmelt further refined that work.[6] In specific, Wineland, Drullinger, and Walls were concerned with the improvement of spectroscopy. The group wrote about experimentally demonstrating the cooling of atoms through a process using radiation pressure. They cite a precedence for using radiation pressure in optical traps, yet criticize the ineffectiveness of previous models due to the presence of the Doppler effect. In an effort to lessen the effect, they applied an alternative take on cooling magnesium ions below the room temperature precedent.[9] Using the electromagnetic trap to contain the magnesium ions, they bombarded them with a laser barely out of phase from the resonant frequency of the atoms.[10] The research from both groups served to illustrate the mechanical properties of light.[6] Around this time, laser cooling techniques had allowed for temperatures lowered to around 40 kelvins.
Modern advancements
William Phillips was influenced by the Wineland paper and attempted to mimic it, using neutral atoms instead of ions. In 1982, he published the first paper outlining the cooling of neutral atoms. The process he used is now known as the Zeeman slower and became one of the standard techniques for slowing an atomic beam. Now, temperatures around 240 microkelvins were reached. That threshold was the lowest researchers thought was possible. When temperatures then reached 43 microkelvins in an experiment by Steven Chu,[11] the new low was explained by the addition of more atomic states in combination to laser polarization. Previous conceptions of laser cooling were decided to have been too simplistic.[10] The major breakthroughs in the 70s and 80s in the use of laser light for cooling led to several improvements to preexisting technology and new discoveries with temperatures just above absolute zero. The cooling processes were utilized to make atomic clocks more accurate and to improve spectroscopic measurements, and led to the observation of a new state of matter at ultracold temperatures.[5][10] The new state of matter, the Bose–Einstein condensate, was observed in 1995 by Eric Cornell, Carl Wieman, and Wolfgang Ketterle.[12]
Doppler cooling
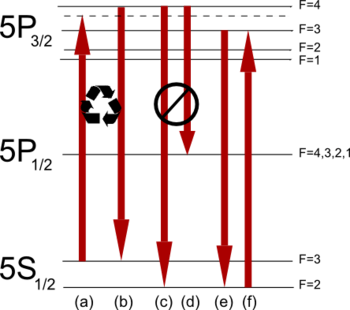
Doppler cooling, which is usually accompanied by a magnetic trapping force to give a magneto-optical trap, is by far the most common method of laser cooling. It is used to cool low density gases down to the Doppler cooling limit, which for rubidium-85 is around 150 microkelvins.
In Doppler cooling, initially, the frequency of light is tuned slightly below an electronic transition in the atom. Because the light is detuned to the "red" (i.e., at lower frequency) of the transition, the atoms will absorb more photons if they move towards the light source, due to the Doppler effect. Thus if one applies light from two opposite directions, the atoms will always scatter more photons from the laser beam pointing opposite to their direction of motion. In each scattering event the atom loses a momentum equal to the momentum of the photon. If the atom, which is now in the excited state, then emits a photon spontaneously, it will be kicked by the same amount of momentum, but in a random direction. Since the initial momentum change was a pure loss (opposing the direction of motion), while the subsequent change was random (i.e., not pure gain), the overall result of the absorption and emission process is to reduce the momentum of the atom, therefore its speed—provided its initial speed was larger than the recoil speed from scattering a single photon. If the absorption and emission are repeated many times, the average speed, and therefore the kinetic energy of the atom, will be reduced. Since the temperature of a group of atoms is a measure of the average random internal kinetic energy, this is equivalent to cooling the atoms.
Uses
Laser cooling is primarily used to create ultracold atoms for experiments in quantum physics. These experiments are performed near absolute zero where unique quantum effects such as Bose-Einstein condensation can be observed. Laser cooling has primarily been used on atoms, but recent progress has been made toward laser cooling more complex systems. In 2010, a team at Yale successfully laser-cooled a diatomic molecule.[13] In 2007, an MIT team successfully laser-cooled a macro-scale (1 gram) object to 0.8 K.[14] In 2011, a team from the California Institute of Technology and the University of Vienna became the first to laser-cool a (10 μm x 1 μm) mechanical object to its quantum ground state.[15]
See also
- List of laser articles – Wikipedia list article
- Optical tweezers
- Zeeman Slower
- Mössbauer effect
- Mössbauer spectroscopy
- Quantum refrigerators
- Timeline of low-temperature technology – aspect of history
- Researchers in laser cooling
- Claude Cohen-Tannoudji – French physicist
- Steven Chu
References
- Laser cooling and trapping of neutral atoms Nobel Lecture by William D. Phillips, Dec 8, 1997: Phillips, William D. (1998). "Nobel Lecture: Laser cooling and trapping of neutral atoms". Reviews of Modern Physics. 70: 721–741. Bibcode:1998RvMP...70..721P. doi:10.1103/RevModPhys.70.721.
- A. Aspect; E. Arimondo; R. Kaiser; N. Vansteenkiste; C. Cohen-Tannoudji (1988). "Laser Cooling below the One-Photon Recoil Energy by Velocity-Selective Coherent Population Trapping". Phys. Rev. Lett. 61 (7): 826–829. Bibcode:1988PhRvL..61..826A. doi:10.1103/PhysRevLett.61.826. PMID 10039440.
- Peter Horak; Gerald Hechenblaikner; Klaus M. Gheri; Herwig Stecher; Helmut Ritsch (1988). "Cavity-Induced Atom Cooling in the Strong Coupling Regime". Phys. Rev. Lett. 79 (25): 4974–4977. Bibcode:1997PhRvL..79.4974H. doi:10.1103/PhysRevLett.79.4974.
- Haller, Elmar; Hudson, James; Kelly, Andrew; Cotta, Dylan A.; Peaudecerf, Bruno; Bruce, Graham D.; Kuhr, Stefan (2015). "Single-atom imaging of fermions in a quantum-gas microscope". Nature Physics. 11 (9): 738–742. arXiv:1503.02005. Bibcode:2015NatPh..11..738H. doi:10.1038/nphys3403.
- Adams and Riis, Charles S. and Erling. "Laser Cooling and Manipulation of Neutral Particles" (PDF). New Optics.
- Phillips, William D. (1998). "Nobel Lecture: Laser cooling and trapping of neutral atoms". Reviews of Modern Physics. 70 (3): 721–741. Bibcode:1998RvMP...70..721P. doi:10.1103/revmodphys.70.721.
- "Cooling of gases by laser radiation - ScienceDirect" (PDF). ac.els-cdn.com. Retrieved 2017-05-05.
- Ashkin, A. (1978). "Trapping of Atoms by Resonance Radiation Pressure". Physical Review Letters. 40 (12): 729–732. Bibcode:1978PhRvL..40..729A. doi:10.1103/physrevlett.40.729.
- Wineland, D. J.; Drullinger, R. E.; Walls, F. L. (1978). "Radiation-Pressure Cooling of Bound Resonant Absorbers". Physical Review Letters. 40 (25): 1639–1642. Bibcode:1978PhRvL..40.1639W. doi:10.1103/physrevlett.40.1639.
- Bardi, Jason Socrates (2008-04-02). "Focus: Landmarks: Laser Cooling of Atoms". Physics. 21. doi:10.1103/physrevfocus.21.11.
- "Laser Cooling". hyperphysics.phy-astr.gsu.edu. Retrieved 2017-05-06.
- Chin, Cheng (2016). "Ultracold atomic gases going strong" (PDF). National Science Review. 3 (2): 168–173. doi:10.1093/nsr/nwv073.
- E. S. Shuman; J. F. Barry; D. DeMille (2010). "Laser cooling of a diatomic molecule". Nature. 467 (7317): 820–823. arXiv:1103.6004. Bibcode:2010Natur.467..820S. doi:10.1038/nature09443. PMID 20852614.
- Massachusetts Institute of Technology (2007, April 8). Laser-cooling Brings Large Object Near Absolute Zero. ScienceDaily. Retrieved January 14, 2011.
- Caltech Team Uses Laser Light to Cool Object to Quantum Ground State. Caltech.edu. Retrieved June 27, 2013. Updated 10/05/2011
Additional sources
- Atomic Physics. Foot, C.J. Oxford University Press (2005). Pdf
- Cohen-Tanoudji, Claude (2011). Advances in Atomic Physics. World Scientific. p. 791. doi:10.1142/6631. ISBN 978-981-277-496-5.
- Bowley, Roger; Copeland, Ed (2010). "Laser Cooling". Sixty Symbols. Brady Haran for the University of Nottingham.
- Laser Cooling HyperPhysics