Chemiresistor
A chemiresistor is a material that changes its electrical resistance in response to changes in the nearby chemical environment.[1] Chemiresistors are a class of chemical sensors that rely on the direct chemical interaction between the sensing material and the analyte.[2] The sensing material and the analyte can interact by covalent bonding, hydrogen bonding, or molecular recognition. Several different materials have chemiresistor properties: metal-oxide semiconductors, some conductive polymers,[3] and nanomaterials like graphene, carbon nanotubes and nanoparticles. Typically these materials are used as partially selective sensors in devices like electronic tongues or electronic noses.
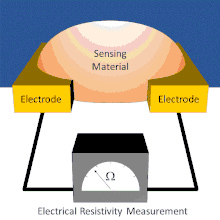
A basic chemiresistor consists of a sensing material that bridges the gap between two electrodes or coats a set of interdigitated electrodes. The resistance between the electrodes can be easily measured. The sensing material has an inherent resistance that can be modulated by the presence or absence of the analyte. During exposure, analytes interact with the sensing material. These interactions cause changes in the resistance reading. In some chemiresistors the resistance changes simply indicate the presence of analyte. In others, the resistance changes are proportional to the amount of analyte present; this allows for the amount of analyte present to be measured.
History
As far back as 1965 there are reports of semiconductor materials exhibiting electrical conductivities that are strongly affected by ambient gases and vapours.[4][5][6] However, it was not until 1985 that Wohltjen and Snow coined the term chemiresistor.[7] The chemiresistive material they investigated was copper phthalocyanine, and they demonstrated that its resistivity decreased in the presence of ammonia vapour at room temperature.[7]
In recent years chemiresistor technology has been used to develop promising sensors for many applications, including conductive polymer sensors for secondhand smoke, carbon nanotube sensors for gaseous ammonia, and metal oxide sensors for hydrogen gas.[2][8][9] The ability of chemiresistors to provide accurate real-time information about the environment through small devices that require minimal electricity makes them an appealing addition to the internet of things.[8]
Types of chemiresistor sensors
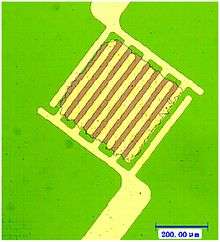
Device architectures
Chemiresistors can be made by coating an interdigitated electrode with a thin film or by using a thin film or other sensing material to bridge the single gap between two electrodes. Electrodes are typically made of conductive metals such as gold and chromium which make good ohmic contact with thin films.[7] In both architectures, the chemiresistant sensing material controls the conductance between the two electrodes; however, each device architecture has its own advantages and disadvantages.
Interdigitated electrodes allow for a greater amount of the film's surface area to be in contact with the electrode. This allows for more electrical connections to be made and increases the overall conductivity of the system.[7] Interdigitated electrodes with finger sizes and finger spacing on the order of microns are difficult to manufacture and require the use of photolithography.[8] Larger features are easier to fabricate and can be manufactured using techniques such as thermal evaporation. Both interdigitated electrode and single-gap systems can be arranged in parallel to allow for the detection of multiple analytes by one device.[11]
Sensing materials
Metal oxide semiconductors
Metal oxide chemiresistor sensors were first commercialized in 1970[12] in a carbon monoxide detector that used powdered SnO2. However, there are many other metal oxides that have chemiresistive properties. Metal oxide sensors are primarily gas sensors, and they can sense both oxidizing and reducing gases.[2] This makes them ideal for use in industrial situations where gases used in manufacturing can pose a risk to worker safety.
Sensors made from metal oxides require high temperatures to operate because, in order for the resistivity to change, an activation energy must be overcome.[2] Typical metal-oxide gas sensors require temperatures of 200 °C or higher in order to operate.[2]
Metal oxide | Vapours |
---|---|
Chromium titanium oxide | H2S |
Gallium oxide | O2, CO |
Indium oxide | O3 |
Molybdenum oxide | NH3 |
Tin oxide | reducing gases |
Tungsten oxide | NO2 |
Zinc oxide | hydrocarbons, O2 |
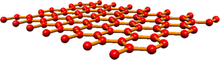
Graphene
In comparison to the other materials graphene chemiresistor sensors are relatively new but have shown excellent sensitivity.[14] Graphene is an allotrope of carbon that consists of a single layer of graphite.[15] TIt has been used in sensors to detect vapour-phase molecules,[16][17][18] pH,[19] proteins,[19] bacteria,[20] and simulated chemical warfare agents.[21][22]
Carbon nanotubes
The first published report of nanotubes being used as chemiresistors was made in 2000.[23] Since then there has been research into chemiresistors and chemically sensitive field effect transistors fabricated from individual single-walled nanotubes,[24] bundles of single-walled nanotubes,[25][26] bundles of multi-walled nanotubes,[27][28] and carbon nanotube–polymer mixtures.[29][30][31][32] It has been shown that a chemical species can alter the resistance of a bundle of single-walled carbon nanotubes through multiple mechanisms.
Carbon nanotubes are useful sensing materials because they have low detection limits, and quick response times; however, bare carbon nanotube sensors are not very selective.[2] They can respond to the presence of many different gases from gaseous ammonia to diesel fumes.[2][9] Carbon nanotube sensors can be made more selective by using a polymer as a barrier, doping the nanotubes with heteroatoms, or adding functional groups to the surface of the nanotubes.[2][9]
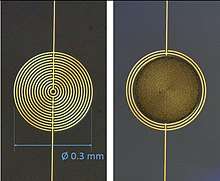
.
Nanoparticles
Many different nanoparticles of varying size, structure and composition have been incorporated into chemiresistor sensors.[33][34] The most commonly used are thin films of gold nanoparticles coated with self-assembled monolayers (SAMs) of organic molecules.[35][36][37][38][39] The SAM is critical in defining some of the nanoparticle assembly’s properties. Firstly, the stability of the gold nanoparticles depends upon the integrity of the SAM, which prevents them from sintering together.[40] Secondly, the SAM of organic molecules defines the separation between the nanoparticles, e.g. longer molecules cause the nanoparticles to have a wider average separation.[41] The width of this separation defines the barrier that electrons must tunnel through when a voltage is applied and electric current flows. Thus by defining the average distance between individual nanoparticles the SAM also defines the electrical resistivity of the nanoparticle assembly.[42][43][44] Finally, the SAMs form a matrix around the nanoparticles that chemical species can diffuse into. As new chemical species enter the matrix it changes the inter-particle separation which in turn affects the electrical resistance.[45][46] Analytes diffuse into the SAMs at proportions defined by their partition coefficient and this characterizes the selectivity and sensitivity of the chemiresistor material.[41][47]
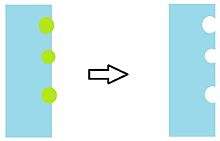
Conductive polymers
Conductive polymers such as polyaniline and polypyrrole can be used as sensing materials when the target interacts directly with the polymer chain resulting in a change in conductivity of the polymer.[8][48] These types of systems lack selectivity due to the wide range of target molecules that can interact with the polymer. Molecularly imprinted polymers can add selectivity to conductive polymer chemiresistors.[49] A molecularly imprinted polymer is made by polymerizing a polymer around a target molecule and then removing the target molecule from the polymer leaving behind cavities matching the size and shape of the target molecule.[48][49] Molecularly imprinting the conductive polymer increases the sensitivity of the chemiresistor by selecting for the target's general size and shape as well as its ability to interact with the chain of the conductive polymer.[49]
References
- Florinel-Gabriel Banica, Chemical Sensors and Biosensors: Fundamentals and Applications, John Wiley and Sons, Chichester, 2012, chapter 11, Print ISBN 978-0-470-71066-1; Web ISBN 0-470710-66-7; ISBN 978-1-118-35423-0.
- Khanna, V.K. (2012). Nanosensors: Physical, chemical, and biological. Boca Raton, FL: CRC Press. ISBN 978-1-4398-2712-3.
- "Archived copy". Archived from the original on 2014-12-17. Retrieved 2014-12-17.CS1 maint: archived copy as title (link)
- J. I. Bregman and A. Dravnieks Surface Effects in Detection, 1965 :Spartan
- F. Gutman and L.E. Lyons Organic Semiconductors, 1967 :Wiley
- Rosenberg, B.; Misra, T. N.; Switzer, R. (1968). "Mechanism of olfactory transduction". Nature. 217 (5127): 423–427. Bibcode:1968Natur.217..423R. doi:10.1038/217423a0. PMID 5641754.
- Wohltjen, H.; Barger, W.R.; Snow, A.W.; Jarvis, N.L. (1985). "A vapor-sensitive chemiresistor fabricated with planar microelectrodes and a langmuir-blodgett organic semiconductor film". IEEE Trans. Electron Devices. 32 (7): 1170–1174. Bibcode:1985ITED...32.1170W. doi:10.1109/T-ED.1985.22095.
- Liu, Yuan; Antwi-Boampong, Sadik; BelBruno, Joseph J.; Crane, Mardi A.; Tanski, Susanne E. (2013-09-01). "Detection of Secondhand Cigarette Smoke via Nicotine Using Conductive Polymer Films". Nicotine & Tobacco Research. 15 (9): 1511–1518. doi:10.1093/ntr/ntt007. ISSN 1462-2203. PMC 3842131. PMID 23482719.
- Azzarelli, Joseph M.; Mirica, Katherine A.; Ravnsbæk, Jens B.; Swager, Timothy M. (2014-12-23). "Wireless gas detection with a smartphone via rf communication". Proceedings of the National Academy of Sciences. 111 (51): 18162–18166. Bibcode:2014PNAS..11118162A. doi:10.1073/pnas.1415403111. ISSN 0027-8424. PMC 4280584. PMID 25489066.
- Wang, H.; Chen, L.; Wang, J; Sun, Q.; Zhao, Y. (2014). "A micro oxygen sensor based on a nano sol-gel TiO2 thin film". Sensors. 14 (9): 16423–33. doi:10.3390/s140916423. PMC 4208180. PMID 25192312.
- Van Gerwen, Peter; Laureyn, Wim; Laureys, Wim; Huyberechts, Guido; Op De Beeck, Maaike; Baert, Kris; Suls, Jan; Sansen, Willy; Jacobs, P. (1998-06-25). "Nanoscaled interdigitated electrode arrays for biochemical sensors". Sensors and Actuators B: Chemical. 49 (1–2): 73–80. doi:10.1016/S0925-4005(98)00128-2.
- Wilson, D. M.; Hoyt, S.; Janata, J.; Booksh, K.; Obando, L. (2001). "Chemical Sensors for Portable, Handheld Field Instruments". IEEE Sensors Journal. 1 (4): 256–274. doi:10.1109/7361.983465.
- Kiani, M. J.; Harun, F. K. C.; Ahmadi, M. T.; Rahmani, M.; Saeidmanesh, M.; Zare, M. (2014). "Conductance modulation of charged lipid bilayer using electrolyte-gated graphene-field effect transistor". Nanoscale Res Lett. 9 (9): 371. doi:10.1186/1556-276X-9-371. PMC 4125348. PMID 25114659.
- Cooper, J. S.; Myers, M.; Chow, E.; Hubble, L. J.; Pejcic, B.; et al. (2014). "Performance of graphene, carbon nanotube, and gold nanoparticle chemiresistor sensors for the detection of petroleum hydrocarbons in water". J. Nanoparticle Res. 16 (1): 1–13. Bibcode:2014JNR....16.2173C. doi:10.1007/s11051-013-2173-5.
- Rao, C.N.R.; Govindaraj, A. (2005). Nanotubes and nanowires. Cambridge, UK: The Royal Society of Chemistry. ISBN 978-0-85404-832-8.
- Schedin, F.; Geim, A. K.; Morozov, S. V.; Hill, E. W.; Blake, P.; et al. (2007). "Detection of Individual Gas Molecules Adsorbed on Graphene". Nature Materials. 6 (9): 652–655. arXiv:cond-mat/0610809. Bibcode:2007NatMa...6..652S. doi:10.1038/nmat1967. PMID 17660825.
- Joshi, R. K.; Gomez, H.; Farah, A.; Kumar, A. (2007). "Graphene Films and Ribbons for Sensing of O2, and 100 ppm of CO and NO2 in Practical Conditions". Journal of Physical Chemistry C. 114 (14): 6610–6613. doi:10.1021/jp100343d.
- Dan, Y.; et al. (2009). "Intrinsic Response of Graphene Vapor Sensors". Nano Letters. 9 (4): 1472–1475. arXiv:0811.3091. Bibcode:2009NanoL...9.1472D. doi:10.1021/nl8033637. PMID 19267449.
- Ohno, Y.; et al. (2009). "Electrolyte-Gated Graphene Field-Effect Transistors for Detecting pH and Protein Adsorption". Nano Letters. 9 (9): 3318–3322. Bibcode:2009NanoL...9.3318O. doi:10.1021/nl901596m. PMID 19637913.
- Mohanty, N.; et al. (2008). "Graphene-Based Single-Bacterium Resolution Biodevice and DNA Transistor: Interfacing Graphene Derivatives with Nanoscale and Microscale Biocomponents". Nano Letters. 8 (12): 4469–4476. Bibcode:2008NanoL...8.4469M. doi:10.1021/nl802412n. PMID 19367973.
- Robinson, J. T.; et al. (2008). "Reduced Graphene Oxide Molecular Sensors". Nano Letters. 8 (10): 3137–3140. Bibcode:2008NanoL...8.3137R. CiteSeerX 10.1.1.567.8356. doi:10.1021/nl8013007. PMID 18763832.
- Hu, N. T.; et al. (2008). "Gas Sensor Based on p-Phenylenediamine Reduced Graphene Oxide". Sensors and Actuators B: Chemical. 163 (1): 107–114. doi:10.1016/j.snb.2012.01.016.
- Kong, J.; et al. (2000). "Nanotube molecular wires as chemical sensors". Science. 287 (5453): 622–5. Bibcode:2000Sci...287..622K. doi:10.1126/science.287.5453.622. PMID 10649989.
- Bradley, K.; et al. (2003). "Short-channel effects in contact-passivated nanotube chemical sensors". Appl. Phys. Lett. 83 (18): 3821–3. Bibcode:2003ApPhL..83.3821B. doi:10.1063/1.1619222.
- Helbling, T.; et al. (2008). "Suspended and non-suspended carbon nanotube transistors for no2 sensing - a qualitative comparison". Physica Status Solidi B. 245 (10): 2326–30. Bibcode:2008PSSBR.245.2326H. doi:10.1002/pssb.200879599.
- Maeng, S.; et al. (2008). "Highly sensitive no2 sensor array based on undecorated single-walled carbon nanotube monolayer junctions". Appl. Phys. Lett. 93 (11): 113111. Bibcode:2008ApPhL..93k3111M. doi:10.1063/1.2982428.
- Penza, M.; et al. (2009). "Effects of reducing interferers in a binary gas mixture on no2 gas adsorption using carbon nanotube networked films based chemiresistors". J. Phys. D: Appl. Phys. 42 (7): 072002. Bibcode:2009JPhD...42g2002P. doi:10.1088/0022-3727/42/7/072002.
- Wang, F.; et al. (2011). "Diverse chemiresistors based upon covalently modified multiwalled carbon nanotubes". J. Am. Chem. Soc. 133 (29): 11181–93. doi:10.1021/ja201860g. hdl:1721.1/74235. PMID 21718043.
- Bekyarova, E.; et al. (2004). "Chemically functionalized single-walled carbon nanotubes as ammonia sensors". J. Phys. Chem. B. 108 (51): 19717–20. doi:10.1021/jp0471857.
- Li, Y.; et al. (2007). "N-type gas sensing characteristics of chemically modified multi-walled carbon nanotubes and pmma composite". Sens. Actuators, B. 121 (2): 496–500. doi:10.1016/j.snb.2006.04.074.
- Wang, F.; et al. (2008). "Carbon nanotube/polythiophene chemiresistive sensors for chemical warfare agents". J. Am. Chem. Soc. 130 (16): 5392–3. doi:10.1021/ja710795k. PMID 18373343.
- Wei, C.; et al. (2006). "Multifunctional chemical vapor sensors of aligned carbon nanotube and polymer composites". J. Am. Chem. Soc. 128 (5): 1412–3. doi:10.1021/ja0570335. PMID 16448087.
- Franke, M.E.; et al. (2006). "Metal and metal oxide nanoparticles in chemiresistors: Does the nanoscale matter?". Small. 2 (1): 36–50. doi:10.1002/smll.200500261. PMID 17193551.
- Ibañez, F.J.; et al. (2012). "Chemiresistive sensing with chemically modified metal and alloy nanoparticles". Small. 8 (2): 174–202. doi:10.1002/smll.201002232. PMID 22052721.
- Wohltjen, H.; et al. (1998). "Colloidal metal-insulator-metal ensemble chemiresistor sensor". Anal. Chem. 70 (14): 2856–9. doi:10.1021/ac9713464.
- Evans, S.D.; et al. (2000). "Vapour sensing using hybrid organic-inorganic nanostructured materials". J. Mater. Chem. 10 (1): 183–8. doi:10.1039/A903951A.
- Joseph, Y.; et al. (2004). "Gold-nanoparticle/organic linker films: Self-assembly, electronic and structural characterisation, composition and vapour sensitivity". Faraday Discussions. 125: 77–97. Bibcode:2004FaDi..125...77J. doi:10.1039/B302678G.
- Ahn, H.; et al. (2004). "Electrical conductivity and vapor-sensing properties of ω-(3-thienyl)alkanethiol-protected gold nanoparticle films". Chem. Mater. 16 (17): 3274–8. doi:10.1021/cm049794x.
- Saha, K.; et al. (2012). "Gold nanoparticles in chemical and biological sensing". Chem. Rev. 112 (5): 2739–79. doi:10.1021/cr2001178. PMC 4102386. PMID 22295941.
- Liu, J.last2=; et al. (2012). "Influence of surface functionalization and particle size on the aggregation kinetics of engineered nanoparticles". Chemosphere. 87 (8): 918–24. Bibcode:2012Chmsp..87..918L. doi:10.1016/j.chemosphere.2012.01.045. PMID 22349061.
- Raguse, B.; et al. (2009). "Gold nanoparticle chemiresistor sensors in aqueous solution: Comparison of hydrophobic and hydrophilic nanoparticle films". J. Phys. Chem. C. 113 (34): 15390–7. doi:10.1021/Jp9034453.
- Terrill, R.H.; et al. (1995). "Monolayers in three dimensions: Nmr, saxs, thermal, and electron hopping studies of alkanethiol stabilized gold clusters". J. Am. Chem. Soc. 117 (50): 12537–48. doi:10.1021/ja00155a017.
- Wuelfing, W.P.last2=; et al. (2000). "Electronic conductivity of solid-state, mixed-valent, monolayer-protected au clusters". J. Am. Chem. Soc. 122 (46): 11465–72. doi:10.1021/ja002367+.
- Wuelfing, W.P.; et al. (2002). "Electron hopping through films of arenethiolate monolayer-protected gold clusters". J. Phys. Chem. B. 106 (12): 3139–45. doi:10.1021/jp013987f.
- Raguse, B.; et al. (2007). "Gold nanoparticle chemiresistor sensors: Direct sensing of organics in aqueous electrolyte solution". Anal. Chem. 79 (19): 7333–9. doi:10.1021/ac070887i. PMID 17722880.
- Müller, K.-H.; et al. (2002). "Percolation model for electron conduction in films of metal nanoparticles linked by organic molecules". Phys. Rev. B. 66 (7): 75417. Bibcode:2002PhRvB..66g5417M. doi:10.1103/Physrevb.66.075417.
- Bohrer, F.I.; et al. (2011). "Characterization of dense arrays of chemiresistor vapor sensors with submicrometer features and patterned nanoparticle interface layers". Anal. Chem. 83 (10): 3687–95. doi:10.1021/ac200019a. PMID 21500770.
- Huang, Jiyong; Wei, Zhixiang; Chen, Jinchun (2008-09-25). "Molecular imprinted polypyrrole nanowires for chiral amino acid recognition". Sensors and Actuators B: Chemical. 134 (2): 573–578. doi:10.1016/j.snb.2008.05.038.
- Antwi-Boampong, Sadik; Mani, Kristina S.; Carlan, Jean; BelBruno, Joseph J. (2014-01-01). "A selective molecularly imprinted polymer-carbon nanotube sensor for cotinine sensing". Journal of Molecular Recognition. 27 (1): 57–63. doi:10.1002/jmr.2331. ISSN 1099-1352. PMID 24375584.