Kinetic Isotope Effects of RuBisCO
The kinetic isotope effect (KIE) of ribulose-1,5-bisphosphate carboxylase oxygenase (RuBisCO) is the isotopic fractionation associated solely with the step in the Calvin-Benson Cycle where a molecule of carbon dioxide (CO
2) is attached to the 5-carbon sugar ribulose-1,5-bisphosphate (RuBP) to produce two 3-carbon sugars called 3-phosphoglycerate (3 PGA). This chemical reaction is catalyzed by the enzyme RuBisCO, and this enzyme-catalyzed reaction creates the primary kinetic isotope effect of photosynthesis.[1] It is also largely responsible for the isotopic compositions of photosynthetic organisms and the heterotrophs that eat them.[2][3] Understanding the intrinsic KIE of RuBisCO is of interest to earth scientists, botanists, and ecologists because this isotopic biosignature can be used to reconstruct the evolution of photosynthesis and the rise of oxygen in the geologic record, reconstruct past evolutionary relationships and environmental conditions, and infer plant relationships and productivity in modern environments.[4][5][6]
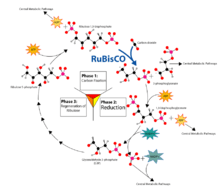
Reaction details and energetics
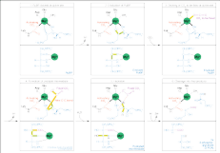
The fixation of CO
2 by RuBisCO is a multi-step process. First, a CO
2 molecule (that is not the CO
2 molecule that is eventually fixed) attaches to the uncharged ε-amino group of lysine 201 in the active site to form a carbamate.[7] This carbamate then binds to the magnesium ion (Mg2+) in RuBisCO's active site. A molecule of RuBP then binds to the Mg2+ ion. The bound RuBP then loses a proton to form a reactive, enodiolate species.[7] The rate-limiting step of the Calvin-Benson Cycle is the addition of CO2 to this 2,3-enediol form of RuBP.[8][9][10] This is the stage where the intrinsic KIE of Rubisco occurs because a new C-C bond is formed. The newly formed 2-carboxy-3-keto-D-arabinitol 1,5-bisphophate molecule is then hydrated and cleaved to form two molecules of 3-phosphoglycerate (3 PGA). 3 PGA is then converted into hexoses to be used in the photosynthetic organism's central metabolism.[7]
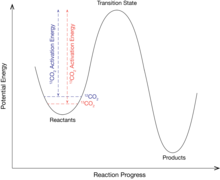
The isotopic substitutions that can occur in this reaction are for carbon, oxygen, and/or hydrogen, though currently only a significant isotope effect is seen for carbon isotope substitution.[11] Isotopes are atoms that have the same number of protons but varying numbers of neutrons. "Lighter" isotopes (like the stable Carbon-12 isotope) have a smaller overall mass, and "heavier" isotopes (like the stable Carbon-13 isotope or radioactive Carbon-14 isotope) have a larger overall mass. Stable isotope geochemistry is concerned with how varying chemical and physical processes preferentially enrich or deplete stable isotopes. Enzymes like RuBisCO cause isotopic fractionation because molecules containing lighter isotopes have higher zero-point energies (ZPE), the lowest possible quantum energy state for a given molecular arrangement.[12] For this reaction, 13CO2 has a lower ZPE than 12CO2 and sits lower in the potential energy well of the reactants. When enzymes catalyze chemical reactions, the lighter isotope is preferentially selected because it has a lower activation energy and is thus more energetically favorable to overcome the high potential-energy transition state and proceed through the reaction. Here, 12CO2 has a lower activation energy so more 12CO2 than 13CO2 goes through the reaction, resulting in the product (3 PGA) being lighter.
Ecological trade-offs influence isotope effects
The observed intrinsic KIEs of RuBisCO have been correlated with two aspects of its enzyme kinetics: 1) Its "specificity" for CO2 over O2, and 2) Its rate of carboxylation.
Specificity (SC/O)
The reactive enodiolate species is also sensitive to oxygen (O2), which results in the dual carboxylase / oxygenase activity of RuBisCO.[13] This reaction is considered wasteful as it produces products (3-phosphoglycerate and 2-phosphoglycolate) that must be catabolized through photorespiration.[14] This process requires energy and is a missed-opportunity for CO2 fixation, which results in the net loss of carbon fixation efficiency for the organism.[13] The dual carboxylase / oxygenase activity of RuBisCO is exacerbated by the fact that O2 and CO2 are small, relatively indistinguishable molecules that can bind only weakly, if at all, in Michaelis-Menten complexes.[15][16] There are four forms of RuBisCO (Form I, II, III, and IV), with Form I being the most abundantly used form. Form I is used extensively by higher plants, eukaryotic algae, cyanobacteria, and proteobacteria.[13] Form II is also used but much less widespread, and can be found in some species of Proteobacteria and in dinoflagellates.[13] RuBisCOs from different photosynthetic organisms display varying abilities to distinguish between CO2 and O2. This property can be quantified and is termed "specificity" (Sc/o). A higher value of Sc/o means that a RuBisCO's carboxylase activity is greater than its oxygenase activity.
Rate of carboxylation (VC) and Michaelis-Menten constant (KC)
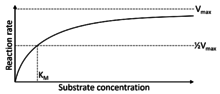
The rate of carboxylation (VC) is the rate that RuBisCO fixes CO2 to RuBP under substrate saturated conditions.[14] A higher value of VC corresponds to a higher rate of carboxylation. This rate of carboxylation can also be represented through its Michaelis-Menten constant KC, with a higher value of KC corresponding to a higher rate of carboxylation. VC is represented by Vmax, and KC is represented as KM in the generalized Michaelis-Menten curve. Although the rate of carboxylation varies among RuBisCO types, RuBisCO on average fixes only three molecules of CO2 per second.[17] This is remarkably slow compared to typical enzyme catalytic rates, which usually catalyze reactions at the rate of thousands of molecules per second.[17]
Phylogenetic patterns
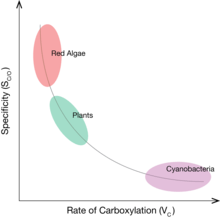
It has been observed among natural RuBIsCOs that an increased ability to distinguish between CO2 and O2 (larger values of Sc/o) corresponds with a decreased rate of carboxylation (lower values of VC and KC)[18]. The variation and trade-off between Sc/o and KC has been observed across all photosynthetic organisms, from photosynthetic bacteria and algae to higher plants.[18] Organisms using RuBisCOs with high values of VC / KC, and low values of Sc/o have localized RuBisCO to areas within the cell with artificially high local CO2 concentrations. In cyanobacteria, concentrations of CO2 are increased using a carboxysome, an icosahedral protein compartment about 100 nm in diameter that selectively uptakes bicarbonate and converts it to CO2 in the presence of RuBisCO.[19] Organisms without a CCM, like certain plants, instead utilize RuBisCOs with high values of Sc/o and low values of VC and KC.[18] It has been theorized that groups with a CCM have been able to maximize KC at the expense of decreasing Sc/o, because artificially enhancing the concentration of CO2 would decrease the concentration of O2 and remove the need for high CO2 specificity. However, the opposite is true for organisms without a CCM, who must optimize Sc/o at the expense of KC because O2 is readily present in the atmosphere.
This trade-off between Sc/o and VC or KC observed in extant organisms suggest that RuBisCO has evolved through geologic time to be maximally optimized in its current, modern environment.[11][18] RuBisCO evolved over 2.5 billion years ago when atmospheric CO2 concentrations were 300 to 600 times higher than present day concentrations, and oxygen concentrations were only 5-18% of present-day levels.[14] Therefore, because CO2 was abundant and O2 rare, there was no need for the ancestral RuBisCO enzyme to have high specificity. This is supported by the biochemical characterization of an ancestral RuBisCO enzyme, which has intermediate values of VC and SC/O between the extreme end-members.[14]
It has been theorized that this ecological trade-off is due to the form that 2-carboxy-3-keto-D-arabinitol 1,5-bisphophate in its transient transition state before cleaving into two 3PGA molecules.[11] The more closely the Mg2+-bound CO2 moiety resembles the carboxylate group in 2-carboxy-3-keto-D-arabinitol 1,5-bisphophate, the greater the structural difference between the transition states of carboxylation and oxygenation.[11] The larger structural difference allows RuBisCO to better distinguish between CO2 and O2, resulting in larger values of Sc/o.[11] However, this increasing structural similarity between the transition state and the product state requires strong binding at the carboxyketone group, and this binding is so strong that the rate of cleavage into two product 3PGA molecules is slowed.[11] Therefore, an increased specificity for CO2 over O2 necessitates a lower overall rate of carboxylation. This theory implies that there is a physical chemistry limitation at the heart of Rubisco's active site, and may preclude any efforts to engineer a simultaneously more selective and faster Rubisco.[11][18]
Isotope effects
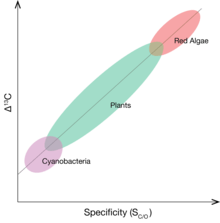
Sc/o has been positively correlated with the magnitude of carbon isotope fractionation (represented by Δ13C), with larger values of Sc/o corresponding with a larger values of Δ13C.[11] It has been theorized that because increasing Sc/o means the transition state is more like the product, the O2C---C-2 bond will be shorter, resulting in a higher overall potential energy & vibrational energy.[11] This creates a higher energy transition state, which makes it even harder for 13CO2 (lower in the potential energy well than 12CO2) to overcome the required activation energy.[11] The RuBisCOs used by varying photosynthetic organisms vary slightly in their enzyme structure, and this enzyme structure results in varying transition states. This diversity in enzyme structure is reflected in the resulting Δ13C values measured from different photosynthetic organisms. However, overlap exists between the Δ13C values of different groups because the carbon isotope values measured are generally of the entire organism, and not just its RuBisCO enzyme. Many other factors, including growth rate and the isotopic composition of the starting substrate, can affect the carbon isotope values of whole organism and cause the spread seen in C isotope measurements.[20][21]
See also
References
- Farquhar, Graham D.; O'Leary, Marion H.; Berry, Joe A. (1982). "On the relationship between carbon isotope discrimination and the intercellular carbon dioxide concentration in leaves". Functional Plant Biology. 9 (2): 121–137. doi:10.1071/PP9820121.
- Zanden, M. Jake Vander; Rasmussen, Joseph B. (November 2001). "Variation in δ15N and δ13C trophic fractionation: Implications for aquatic food web studies". Limnology and Oceanography. 46 (8): 2061–2066. Bibcode:2001LimOc..46.2061Z. doi:10.4319/lo.2001.46.8.2061. ISSN 0024-3590.
- McCutchan, James H.; Lewis, William M.; Kendall, Carol; McGrath, Claire C. (August 2003). "Variation in trophic shift for stable isotope ratios of carbon, nitrogen, and sulfur". Oikos. 102 (2): 378–390. doi:10.1034/j.1600-0706.2003.12098.x. ISSN 0030-1299.
- Farquhar, G. D.; Hubick, K. T.; Condon, A. G.; Richards, R. A. (1989), "Carbon Isotope Fractionation and Plant Water-Use Efficiency", Stable Isotopes in Ecological Research, Springer New York, pp. 21–40, doi:10.1007/978-1-4612-3498-2_2, ISBN 9781461281276
- Körner, Ch.; Farquhar, G. D.; Wong, S. C. (September 1991). "Carbon isotope discrimination by plants follows latitudinal and altitudinal trends". Oecologia. 88 (1): 30–40. Bibcode:1991Oecol..88...30K. doi:10.1007/bf00328400. ISSN 0029-8549. PMID 28312728.
- Hayes, John M.; Strauss, Harald; Kaufman, Alan J. (September 1999). "The abundance of 13C in marine organic matter and isotopic fractionation in the global biogeochemical cycle of carbon during the past 800 Ma". Chemical Geology. 161 (1–3): 103–125. Bibcode:1999ChGeo.161..103H. doi:10.1016/s0009-2541(99)00083-2. ISSN 0009-2541.
- Berg JM, Tymoczko JL, Stryer L (2012). Biochemistry (7th ed.). New York: W.H. Freeman. ISBN 9781429229364. OCLC 758952268.
- Chen Z, Spreitzer RJ (March 1991). "Proteolysis and transition-state-analogue binding of mutant forms of ribulose-1,5-bisphosphate carboxylase/oxygenase from Chlamydomonas reinhardtii". Planta. 183 (4): 597–603. doi:10.1007/BF00194282. PMID 24193854.
- Cleland WW, Andrews TJ, Gutteridge S, Hartman FC, Lorimer GH (April 1998). "Mechanism of Rubisco: The Carbamate as General Base". Chemical Reviews. 98 (2): 549–562. doi:10.1021/cr970010r. PMID 11848907.
- Pierce J, Andrews TJ, Lorimer GH (August 1986). "Reaction intermediate partitioning by ribulose-bisphosphate carboxylases with differing substrate specificities". The Journal of Biological Chemistry. 261 (22): 10248–56. PMID 3090034.
- Tcherkez GG, Farquhar GD, Andrews TJ (May 2006). "Despite slow catalysis and confused substrate specificity, all ribulose bisphosphate carboxylases may be nearly perfectly optimized". Proceedings of the National Academy of Sciences of the United States of America. 103 (19): 7246–51. Bibcode:2006PNAS..103.7246T. doi:10.1073/pnas.0600605103. PMC 1464328. PMID 16641091.
- Eiler, John M. (October 2007). ""Clumped-isotope" geochemistry—The study of naturally-occurring, multiply-substituted isotopologues". Earth and Planetary Science Letters. 262 (3–4): 309–327. Bibcode:2007E&PSL.262..309E. doi:10.1016/j.epsl.2007.08.020. ISSN 0012-821X.
- Tabita FR, Hanson TE, Satagopan S, Witte BH, Kreel NE (August 2008). "Phylogenetic and evolutionary relationships of RubisCO and the RubisCO-like proteins and the functional lessons provided by diverse molecular forms". Philosophical Transactions of the Royal Society of London. Series B, Biological Sciences. 363 (1504): 2629–40. doi:10.1098/rstb.2008.0023. PMC 2606765. PMID 18487131.
- Shih PM, Occhialini A, Cameron JC, Andralojc PJ, Parry MA, Kerfeld CA (January 2016). "Biochemical characterization of predicted Precambrian RuBisCO". Nature Communications. 7: 10382. Bibcode:2016NatCo...710382S. doi:10.1038/ncomms10382. PMC 4735906. PMID 26790750.
- Halliwell B, Stumpf PK, Conn EE, Hatch MD, Boardman NK (1982-02-08). "The Biochemistry of Plants: A Comprehensive Treatise". FEBS Letters. 138 (1): 153. doi:10.1016/0014-5793(82)80429-8.
- Pierce J, Lorimer GH, Reddy GS (1986). "Kinetic mechanism of ribulosebisphosphate carboxylase: Evidence for an ordered, sequential reaction". Biochemistry. 25 (7): 1636–1644. doi:10.1021/bi00355a029.
- "PDB101: Molecule of the Month: Rubisco". RCSB: PDB-101. Retrieved 2018-05-25.
- Savir Y, Noor E, Milo R, Tlusty T (February 2010). "Cross-species analysis traces adaptation of Rubisco toward optimality in a low-dimensional landscape". Proceedings of the National Academy of Sciences of the United States of America. 107 (8): 3475–80. arXiv:1007.4461. Bibcode:2010PNAS..107.3475S. doi:10.1073/pnas.0911663107. PMC 2840432. PMID 20142476.
- Yeates TO, Kerfeld CA, Heinhorst S, Cannon GC, Shively JM (September 2008). "Protein-based organelles in bacteria: carboxysomes and related microcompartments". Nature Reviews. Microbiology. 6 (9): 681–91. doi:10.1038/nrmicro1913. PMID 18679172.
- Laws, Edward A.; Popp, Brian N.; Bidigare, Robert R.; Kennicutt, Mahlon C.; Macko, Stephen A. (March 1995). "Dependence of phytoplankton carbon isotopic composition on growth rate and [CO2)aq: Theoretical considerations and experimental results". Geochimica et Cosmochimica Acta. 59 (6): 1131–1138. Bibcode:1995GeCoA..59.1131L. doi:10.1016/0016-7037(95)00030-4. ISSN 0016-7037.
- Popp, Brian N.; Laws, Edward A.; Bidigare, Robert R.; Dore, John E.; Hanson, Kristi L.; Wakeham, Stuart G. (January 1998). "Effect of Phytoplankton Cell Geometry on Carbon Isotopic Fractionation". Geochimica et Cosmochimica Acta. 62 (1): 69–77. Bibcode:1998GeCoA..62...69P. doi:10.1016/s0016-7037(97)00333-5. ISSN 0016-7037.